Abstract: Human gene therapy research is currently discouraging due to the lack of suitable delivery vehicles for nucleic acid transfer to affected cell types.
There is an urgent need for optimized gene delivery tools capable of protecting the polynucleotide from degradation through its route from site of administration to gene expression.
Besides difficulties arising during the preparation of the currently employed cationic lipids, their cytotoxicity has been an unavoidable hurdle. Some energetics issues related to preparation and use of self-assemblies formed between neutral lipid and polynucleotides with various conformation and size are presented.
The divalent metal cation-governed adsorption, aggregation and adhesion between single- and double-stranded polynucleotides with multilamellar and unilamellar phosphatidylcholine vesicles was followed turbidimetrically. Thermotropic phase transitions of zwitterionic liposomes and their complexes with polynucleotides and calf thymus DNA with Ca2+ and Mg2+ is presented and compared to the previous data for various electrostatic lipid - nucleic acid complexes.
Differential scanning microcalorimetric measurements of synthetic phosphatidylcholine vesicles and polynucleotides and their ternary complexes with inorganic cations were used to build the thermodynamic model of their structural transitions. The increased thermal stability of the phospholipid bilayers is achieved by affecting their melting transition temperature by nucleic acid induced electrostatic charge screening. Thermodynamic measurements give evidence for the stabilization of polynucleotide helices upon their association with liposomes in presence of divalent metal cations. It is thus possible to suggest this self-assembly as an improved formulation with further potential in gene therapy trials. Although the pharmacodynamical features of the zwitterionic lipid-metal ion-DNA nanocondensates remain to be tested in further transfection experiments, at least from physicochemical viewpoint, stability parameters are encouraging to consider them as a promising metal-based nucleic acid pharmaceuticals.
Keywords: Zwitterionic lipid - polynucleotide self - assemblies; divalent metal cations; differential scanning microcalorimetry; phase transitions; non-viral gene delivery
INTRODUCTION
Spontaneously formed self-organized systems comprise the multidisciplinary research interest. Beside the biologically inspired interest in fundamental property of the living matter to form self-assembled assemblages, they are approached by physical chemists, as well, in terms of pure supramolecular reasoning.
These structures are often investigated to test proposed colloidal and physicomathematical models. Thus, the idea have been to build various complexes between smaller substrates and their larger guests (host-guest complexes). Numerous host-guest complexes were studied at different levels and viewpoints1 . Thus, ions and larger charged molecules were non-specifically complex or conjugated with oppositely charged or neutral species. Subsequently, their relevant natural counterparts were searched for. From generally encountered basic types of biomacromolecule associates-lipids, polysaccharides, proteins and nucleic acids, principally six types of covalent and non-covalent pairs of complexes can be formed.
However, very few were described so far. Interestingly, no any complex formed between lipids and nucleic acids is investigated in depth, and the current knowledge concerning them at in vivo level is insufficient. This assembly is reported only for isolated molecules in organic solvents, which are the subject of preparative biochemistry. Cellular events, however occur in macromolecularly crowded surroundings. Hence, lipid complexes with nucleic acids have been regarded as artificial laboratory or microscopy preparations.
Researchers from the origin of life field considered them as a primitive cellular forms in a RNA-dominated primordial soup. Subsequently, such self-assemblies have been looked for in crucial cellular events. In prokaryotes, example of such aggregate is mesosome, which itself has been considered as an accidental artifact formed during specimen preparation for microscopy, or another macromolecular biopolimers occurring during bacterial nucleoid packaging and segregation. mRNA maturation and transport through nuclear pores, cell division and phage infection are the examples of nucleic acid-lipid interactions among eukaryotes. Attempts to isolate this labile complexes in vivo currently face certain problems. The difficulty of describing these complexes at the cellular level are their instability, in relation to their kinetics of formation.
Recently, the research interest on interactions between lipids and both DNA and RNA of different sizes and conformations has arisen after realization of their potential for employment as gene carriers in human gene therapy. Despite voluminous research reports on nucleic acid aggregation with liposomes of various lipid composition, the colloidal factors and forces governing their complex formation remain to be understood. Additional requirements of size homogeneity, stability, ability to keep the entrapped therapeutic gene sequence in sufficient concentration and reproducible manufacturing issues render the development of such gene carriers difficult. Thus, clinical trials indicate that little has been achieved concerning efficiency for the patients. Making reliable viral vectors is highly problematic. Attention has switched to construct a safer non-viral alternative. Currently, polycation- and lipid complexes with DNA, or combinations between them, have been developed as promising non-viral gene therapeutic vehicles.
Therapeutic gene transfer is regarded as employment of polynucleotide sequences as medicines to cure disease, or more specifically is referred to as nucleic acid delivery to patients for subsequent clinical benefit2-7. This goal is achieved by using viral or non-viral, synthetic or physical methods. The latter uses mainly electroporation protocols, which are well-described mathematically. However, electric field induced DNA transfer face problems with electrodeformation of the nucleic acid, physical entry of DNA into the desired cell type and show high dependence on ionic conditions employed in the experimental design8.
The clinically well-established viral-based delivery vectors5,9 are superior in terms of efficiency compared to other transgene carriers. These vehicle constructs include a variety of mamalian, insect (e. g. baculovirus expression systems) and bacteriophage constucts. Both highly successful (adenoviral vectors) and those possessing poor gene delivery capabilities (e. g. retroviral constructs) are known5. The major concern regarding viral-based gene delivery is due to their immunotoxic side effects5,9. In this context, it is widely believed that non-viral gene therapy will surpass viral delivery systems in time. Non-viral nucleic acid therapy includes complexes obtained from DNA-synthetic polycations (polyplexes)1,10,11, or DNA-lipid (lipoplexes) mixtures12. The objective is to increase the transgene expression, with improved bioavailability and decreased toxicity of both carrier systems.
Once formed, polyplexes or lipoplexes should enter the desired cells for further expression of the therapeutic gene activity (Fig. 1). The route shown on Fig. 1 involves numerous well-documented problems5-7. Thus, both particles often aggregate in serum much before approaching the target site. Moreover, both of these unfortunately heterogeneous nanometric structures are neither cell-type specific nor able to enter cell interior within desirable time. Once inside the cell, further stability and aggregation problems continue. Despite the initial hope that polyplexes can escape degradation by late endosomes, current laboratory data indicates the frequent occurrence of the opposite effect.
Fig. 1. A current view of entry routes of lipoplexes in target cell. Three mechanisms referred to as receptor-mediated endocytosis, lipid-mediated poration and lipid fusion are depicted to show the hurdles for efficient transfection. Original drawing by Dr. D. E. Hansen (NIH).
Since lipid-based systems are preferred to polyplexes (http://www.wiley.co.uk/genetherapy/clinical/) our research is focused on the well-established potential of liposomes2,4,13as gene carriers. As reviewed recently6,7, synthetic non-viral vectors have numerous advantages compared with viral carriers. They show lower immunogenicity with diminished potential for oncogenicity. Nucleic acids with a variety of sizes, ranging from short oligonucleotides to artificial chromosomes, can be delivered. Moreover, they permit performance of simpler quality controls, as well as easier satisfaction of pharmaceutical prerequisites6.
The currently built models on DNA-lipid systems are based on research devoted to initial biophysical and ultrastructural characterization of either plasmid DNA or another model nucleic acid with mostly cationic lipids followed with a transfection assay to test their efficiency for intracellular transport in cell cultures. We have focused more on synthetic lipids than the nucleic acid itself. Following our current approach to consider poly(ribo)nucleotide-(phospho)lipid self-assemblies both from above mentioned cell biological and practical viewpoints, it is interesting to develop constructs of smaller oligo- and polynucleotides, as well as other nucleic acids as complexes with lipids.
In our opinion, such studies will open new insights into unravelling the yet undescribed nucleic acid receptors on cell surfaces with implications in development of certain pathologies14. On the other hand, the recent emphasis on use of oligonucleotides in gene delivery studies need primarily biophysical stability data on their complexes with lipid carriers15.
Viruses have an evolutionary driven uncoparably higher capacity for transfection compared to non-viral agents. They achieve this by destabilizing the host cell membrane via viral fusion followed by cellular penetration of nucleic acids. This is thought to be DNA size-dependent and electrostatically controlled event. The exact nature, properties and way of action of molecules that can destabilize cell membrane is unclear. Hence, one approach to improve transfection efficiency of therapeutic gene carriers is to mimic the way of action of viruses. In designing novel synthetic viral nucleic acid delivering systems, through such a membrane fusion pattern, instead of receptor mediated pathway, it is important to consider the more naturally encountered electrified surfaces.
The present study reports some new results concerning kinetic and thermodynamic parameters of phospholipid-nucleic acid array formations. The objective is to describe initial complexation features of these organized molecular systems, emphasizing the use of zwitterionic species as alternatives of the cytotoxic cationic lipids, as also suggested previously by Kharakoz et al (1999)16.
Literature survey shows that usually laboratory protocols use simply addition of DNA to liposome suspension, with their subsequent mixing in various lipid/DNA ratios. Since, the latter is highly dependent on the nucleic acid and liposome size and type, instruments used and experimental designs, the reported parameters are often speculative and contradictory to each other.
Hence, our aim was also to compare our data with previous measurements for optimization of our currently employed laboratory setup. Our efforts are focused on nanoaggregates formation between liposomes and DNA, mediated by inorganic cations, condensing the whole complex. The motivation for this particular design comes from recent reports on positive effects of Ca2+ on tranfection efficiency17. The particular properties of phosphatidylcholine vesicles' complexation with polynucleotides and calf thymus DNA in the presence of Mg2+, are reported. More specifically, Mg2+ was chosen as a condensing agent, due to its role in regulating various cellular functions. Having considered the high physiological concentration of Mg2+ intracellularly, ADP and ATP exist mainly as their Mg2+-complexes, which has further implications in enzymatic reactions utilizing hydrolysis or synthesis of ATP. Moreover, Mg2+ is an tremendous agent for phosphate group transfers18, making it a preferable naturally occurring element compared to currently employed synthetic polymers, which are not encountered in biofluids.
On the other hand, the transport of ions occurs partly via metal binding to amphiphilic membranes. Since phosphatidylcholine is generally a major member of the total natural phospholipid bilayers, it is important to study the interaction with a variety of metals. Thus, employing Mg2+-phosphatidylcholine system would give further data on biological importance of metal ion control of biomembrane fluidity. Relevant comparisons with Ca2+ and related neutral lipids are mentioned at appropriate points as well. Since DNA transfer and cellular internalization are size dependent processes, the use of polynucleotide species of different size, base composition and chain conformation is emphasized.
MATERIALS AND METHODS
Materials
Synthetic polyriboadenylic acid: polyribouridilic acid (poly(A:U)n), polyriboguanylic acid: polyribocytidylic acid (poly(G:C)n) duplexes (200 kDa each) polycytidilic acid (poly(C)n), 1,2-dimyristoyl-sn-glycero-3- phosphatidylcholine (DMPC) and 1,2-dipalmitoyl-sn-glycero-3-phosphatidylcholine (DPPC), solid HEPES, SSC and TRIS buffer reagents were purchased from Sigma (St. Louis, MO, USA) and used without further purification. All other reagents were of analytical grade.
Methods
Preparation of Polynucleotide Solutions and Concentration Determinations
Four different polynucleotides (SIGMA) were dissolved in buffer. Concentrations of single- and double-stranded polynucleotides were determined spectrophotometrically by using the molar extinction coefficients per base pair, as employed previously19. During all kinetic and calorimetric experiments, polynucleotide concentrations were 0.14 mg/1 ml 10 mM particular buffer/10 mM NaCl, pH=7.22.
Calf thymus DNA with MW of 8.6 MDa (= 13 kb), 42% GC, Tm = 87°C, ~20 A260 units per mg DNA was used. Polinucleotide concentrations and molar ratios are based on the average nucleotide molecular weight of 308 20.
Preparation of liposomes
Chromatographic tests for purity of the lipids were not performed, however the purity of the lipid preparation was assured from the half-widths of their main phase transitions21. 1.2 mM lipid in standard SSC, HEPES and phosphate buffer, pH=7.2 was used in all experiments and was stored at 4°C.
The formation of a thin layer of lipids of a 15 ml round-bottomed flask was achieved by a hand-shaking and hydration in particular buffer at around 70°C. Vortexing of the lipid with the desired aqueous solution above the gel-to-liquid crystalline phase transition of the lipid (Tm) for around 30 min resulted in multilamellar vesicles.
The DNA concentration used throughout all experiments was 1.8 mM based on the above mentioned assumption.
Unilamellar vesicles were obtained by extrusion of multilamellar vesicle suspension through two stacked polycarbonate filters (Nucleopore, Inc.) of 100 nm pore size at around 60°C. Repeated extrusion (10 times) through the extruder (Lipex Biomembranes, Inc., Vancouver, B. C., Canada) created homogeneous vesicle suspension. This allowed the preparation of vesicles with a mean diameter of 90 nm and a trap volume in the range of 1.5 – 2.0 l/mole.
Preparation of liposome-nucleic acid mixtures
Nucleic acid-lipid mixtures were prepared 1 hour before turbidimetric measurements by vigorous mixing of either phosphatidylcholine MLV or ULV dispersions and solvent, varying nucleic acid concentration and keeping DPPC concentration fixed. Control experiments of DNA-lipids in the absence of divalent cations, DNA-metal ions, and DPPC-metal ions were performed in parallel. Lipid vesicles' concentration was 0.3 mg/ml.
The lipid samples were hydrated, as described above to form first MLV. The preparation of phosphatidylcholine ULV-polyribonucleotide duplexes, or with calf thymus DNA, was the same as in the case of MLVs, i.e. by mixing DNA solution with aqueous DPPC dispersion in the presence of Mg2+ or Ca2+.
The DNA concentration used throughout all experiments was 1.8 mM based on the above mentioned assumption. A freeze-thaw protocol was followed to ensure equal distribution of solutes between lamellae and adequate hydration of the lipids.
Comparison with the case of liposomal preparations without employing freeze-thaw procedure showed no difference in terms of homogeneity of the suspension. This was done by placing the sample in a cryo-tube and freezing it in liquid nitrogen for around 30 sec. The cryo-tube was subsequently removed and was plunged into warm water (~60˚C). When the sample was thawed, the whole cycle of freeze-thawing was repeated 6 times.
UV/VIS Spectrophotometry
The concentration of DNA was checked from ultraviolet (UV) absorption at 260 nm using the relation 1.0 absorbance unit (A)=50 µg/ml nucleic acid. The whole spectrum was scanned for lipids, and the maximum absorption peak value was chosen as a wavelength for further measurements.
While these measurements were performed at constant temperature of 25±2˚C, the kinetics of metal cation induced complexation between polynucleotides and liposomes were recorded at different temperatures both below and above their phase transition temperature in order to follow the DNA-induced change of lipid fluidity.
The spectra of phospholipids and polynucleotides alone, or their combinations in the presence of Mg2+ were recorded with Shimadzu A160 double beam spectrophotometer (Schimadzu Co. Ltd., Japan) using 3 ml quartz cuvettes thermostatized within ±0.3˚C by circulating water bath connected to the cuvette holder.
Temperature inside the cuvette was controlled by a thermocouple. For this, the reference cuvette was thermostatted and the measurement cuvette heated, automatically recording change in optical density at the constant rate (2±0.2˚C).
The fluctuation of the parameters of the helix-to-coil transition of polynucleotides due to imbalance of chain melting did not exceed statistically significant limits (0.6˚C)22.
Linear heating and cooling of the samples was performed at constant rates using distilled water as a standard, as described21.
The absorption spectra of polynucleotides and lipids were separated from each other by simultaneously performing the measurements at their corresponding wavelengths, respectively23.
Turbidimetric Measurements
The preferred method for following polynucleotide adsorption and adhesion on liposome surfaces leading to electrostatically driven formation of larger aggregates is employment of turbidity measurements. This method is more suitable than the light scattering determinations, since there is no angular dependence involved, occurring for solutions of liposomes with diameters greater than ~20 nm, or ~1/20th of the wavelength of incident beam. Hence, wavelength corrections due to light scattering are not needed24,25.
The kinetics of polynucleotides with zwitterionic vesicles in the presence of Mg2+ was followed turbidimetrically at both below and above lipid phase transition temperature, as described above.
To avoid turbid solutions occurring during the lengthy determinations, two wavelength measurements were performed, which showed the same trend. Care was taken to prevent precipitation of MLVs during these assays by loading them with 16.2% sucrose solution.
The scheme of kinetics of divalent metal cation triggered complexation of polynucleotides with phospolipid vesicles is shown on Fig. 2. The aggregation of the three molecular species during the first 5-10 min. was followed, since prolonged assays did not result in any further significant increase in turbidity. Divalent metal cations were added at the 30th sec. of the initial polynucleotide-MLV or ULV binary mixture with subsequent stirring of the cuvette.
Fig. 2. Aggregation and kinetics of adsorption of DPPC-MLV and ULV with poly(ribo)nucleotides in the presence of divalent metal cation (Me2+) at constant temperature at 550 nm. After their preparation, both MLV and ULV were left for 1 hour. Their initial absorbance vary with respect to each other due to their different lamellarity, curvature and homogeneity. The stock solutions of poly(ribo)nucleotides and calf thymus DNA were prepared freshly and used immediately before measurements. After zeroing of the initial apparent absorption, their complexation with lipid vesicles was followed during the first 30 sec. Metal ions (Me2+) were added to the binary mixture of polynucleotide and liposomes at the final concentration of 0.5 mM and the mixture was mixed in the cuvette. The formed triple biopolymer complex is destroyed by EDTA treatment. The evaluation of their ternary complex formation was performed, as outlined in Materials and Methods.
The increase in turbidity of the aggregate after addition of the metal cations were plotted on chart recorder and used afterwards for estmations of statistical significance. Aggregation rate constants were calculated from the initial slopes of the turbidity (τ) as a function of time (t)26.
(1/t0)(dt/dt)0
k11=___________________
[(C2/2C1)-1]N1
&where k11 refers to the rate constant of doublet formation, t0 is the initial turbidity, N1 the number concentration, and [(C2/2C1)-1] is the optical factor. All terms in this equation were substituted with relevant values, according to Gamon et. al., (1989)26. The optical factor was determined using Rayleigh-Gans-Debye light scattering theory with the appropriate factor for spherical shells, in agreement with the treatment of Chong C. S. and Colbow, K. (1976)24.
The data obtained was evaluated over a range of metal chlorides, polynucleotides and lipid concentrations. A total of 6 turbidity measurements per each temperature mentioned was performed and the plotted results were averaged. However, instead of using the stability ratio, W, for description of aggregation rates, in terms of Smoluchowski theory, we used another parameter (α), solely for comparative purposes with other works on phospholipid-inorganic metal cation-DNA ternary complexes27.
The physical parameter a was proposed originally by Dr. V. Budker (Institute of Bioorganic Chemistry, Siberian Branch of The ex-Soviet Academy of Sciences-Novosibirsk) and used as a constant to determine the degree of aggregation and adhesion of phospholipid vesicles with poly(ribo)nucleotides with or without metal cations27, as the ratio:
α=Apolynucleotides-Mg2+- lipid vesicles /Apolynucleotides-lipid vesicles,
where A is the apparent absorption. This is therefore a ratio of absorbance values measured after addition of divalent metal cations to that before complexation with inorganic cations.
Differential Scanning Calorimetry
Calorimetric measurements were performed using Privalov type high sensitivity differential adiabatic scanning microcalorimeter DASM-4 (Biopribor, Pushchino, Russia) with sensitivity higher than 4.10-6 cal K-1 and a noise level less than 5.10-7 W28. Heating runs were performed with a scan rate of 0.5 K/min. The temperature at the maximum of the excess heat capacity curve was taken as the transition temperature Tm and the transition width ∆T1/2 was determined at the transition half-height. The specific heat capacity of the lipids Cp(lip)(T) was calculated from the relationship21:
where Cp, app water (T) and Vapp water (T) are the apparent specific heat capacity and volume of water taken from Handbook of Chemistry and Physics, 66th Edn. (1985-86), ∆Cp, obs (T) is the observed difference in heat capacity between the lipids and the water baseline, Vapplip (T) is the apparent specific volume of lipid and is given by published densitometric values, and mlip is the mass of the employed lipid. The calorimetric enthalpy ∆Hcal of the transition was determined as the area under the excess heat capacity curve. The van't Hoff enthalpy ∆HvH was calculated from the calorimetric data by using the relationship21:
where Cpmax is the maximum excess heat capacity.
Care was taken to ensure the reproducibility of the obtained result in terms of instrumental drift. For this, microcalorimetric measurements of melting behaviour of lipids in complex with poly(A:U)n and calf thymus DNA in various lipid/DNA ratios were carried out using another instrument (SETARAM DSC microcalorimeter, Caluire, France), equiped with Hewlett-Packard PC and with company supplied computer programme. Scanning rate of 0.5 or 1.0°C/min and scanning range between 17°C and 95°C was used throughout measurements. Amplification range was 0.250 mV with 1500 points.
RESULTS AND DISCUSSION
The design of nucleic acid delivery formulations currently proceeds as searches of alternatives to highly risky viral vectors. In this context, the emphasis is put more on formulating more effective delivery systems rather than on engineering therapeutic genes, which itself will take many years, indicating the fact that gene therapy is a high risk commercially. The objective of liposomal delivery of nucleic acids is to achieve compaction of genetic material within highly restricted lipid compartments, while decreasing its toxicity. Hence, the stability and physical properties of DNA within the lipid surrounding is of major concern.
These characteristics of nucleic acids are well documented in various model membrane systems, such as Langmuir-Blodgett (LB) films, black lipid membranes (BLM) and liposomes. While the first two methods provide evidence for surface adsorption and penetration of DNA through lipid mono- and bilayers, liposomes are preferred subject due to their oval form thus approaching three-dimensionally the cell shapes.
The possibility of creating a variety of sizes, electrostatic and hydrophobic forces and therefore controlling vesicle curvatures are few factors making liposomes attractive as gene delivery carriers. Since, the stability of the liposome-nucleic acid formulations is detrimental factor for subsequent cellular studies, the first contacts between nucleic acids and lipids, namely, adhesion, aggregation of DNA onto liposomes, and energetics of cation-induced complex formation between these biopolymers, are emphasized.
The present work describes preliminary measurements on poly(ribo)nucleotide-zwitterionic liposome self-assembly formation as a possible alternative of the currently employed problematic cationic lipids in gene delivery. The results on coplexation between DPPC-MLV and ULV and polynucleotides in the presence of Mg2+ are presented for brevity. Comparisons with DMPC and Ca2+ are mentioned as well.
The majority of studies on lipid vesicles' aggregation deal with well-established electrostatic reasoning on inorganic cation effects on negatively charged lipids. Few works devoted to cation binding to neutral phospholipids report difficulties with determinations of the rate constants, especially when varying temperature. Overcoming the discrepancy of the reported rate constants is a prerequisite for verifying the existing mechanistic theories.
In this, study, we seek particularly to test the concept of a hydration forces as an explanation of the observed complexations. The aggregation of liposomes with polynucleotides and larger DNAs in the presence of Mg2+ can be observed by optical microscopy. However, applying spectroscopic measurements have the following advantages. Light scattering methods have been widely used in liposome research, to determine shapes and sizes of phospholipid dispersions24,25.
The theory of light scattering and turbidity methods as applied for observation of thermotropic phase transitions in both natural and artificial membranes is given by Chong, C. S. and Colbow, K. (1976)24. The application of UV/VIS spectroscopy, used also in the current study, in gene delivery studies was recently reviewed29.
Briefly, in comparison with electron microscopy, the use of light scattering measurements is more suitable, since the system can be observed without large perturbations, while the sizes and shapes of vesicles visualized in electron micrographs often depend on the fixing or staining method employed, thus creating preparation artifacts. The scattered light can be observed at a right angle to the incident beam24,25. Turbidity (τ) is the decrease by scattering of the transmitted light, and thus is equal to the scattering over all angles.
Having also considered the above mentioned lack of angular dependence of liposomes, the preferred method is spectrophotometric measurement of the intensity of the transmitted beam instead of using light scattering changes.
The complexations between poly(ribo)nucleotides with different conformation and neutral phosphatidylcholine vesicles were studied with emphasis on the kinetics of the associations. Fig.2 shows the turbidity changes of their ternary associations with divalent metal cations. No any turbidity was detected in poly(ribo)nucleotide samples in the absence of metal cations.
As seen, their associations with liposome vesicles do not lead to any turbidity change as well. After addition of metals and rapidly mixing of the cuvette content, a substantial increase of turbidity of the suspension is seen, indicating the formation of larger aggregates. Ca2+-induced aggregation between polynucleotide helix and liposomes is slightly higher as compared to Mg2+. The physical parameter α was used as a criteria for evaluation of the ternary complex formation of these biopolymers (Table 1 and 2). MLVs showed no any turbidity increase in the presence of metal caions. Turbidity changes were observed only with ULVs.
This result suggests that interlamellar repulsive forces are stronger than the exerted effect of the inorganic cations. This is an example of the influence of cation strength and valency on the structure and size of collapsed DNA. Apparently, this complexation type is valency-dependent, the effect being stronger in the case of at least three positive charges on it. The formed toroidal DNA molecule is more affected than lipids. This DNA aggregates with several MLVs, with very little tendency to penetrate at least partly to their intramolecular spaces (Fig.5).
The chosen poly(ribo)nucleotides and calf thymus DNA permit testing the effect of chain conformation on complexation pattern. Interestingly, the single-stranded polycytidylic acid showed different complexation behaviour, when taken in various concentrations. Thus, when used in doubled concentration, this homopolynucleotide showed the greatest value of the α parameter.
The effect is due to the base-stacking properties of poly(C) n when taken in high amounts30. This is a case of complexation occurring without vesicles’ aggregation and the α parameter remains constant, indicating a possible adhesion of this synthetic poly(ribo)nucleotide onto the vesicle surface. On the other hand, poly(G:C)n showed higher value of α parameter, as compared to poly(A:U)n, indicating the effect of H-bond effect. Thus, the observed aggregation trend of poly(ribo)nucleotides was:
αpoly(C)n ~α calf thymus DNA <α poly(A:U)n <α poly(G:C)n
and is essentially concentration-dependent. This complexation behaviour is maintained both below and above phase-transition temperature of the lipids. Parallel experiments with closely related lipid DMPC showed the same trend. DMPC and DPPC differ only slightly in structure, but have different Tm. Thus, DMPC possess greater fluidity than DPPC. The fluidity of lipids, however, did not affect the complexation pattern of the ternary associations (Table 1 and 2).
Table 3 is a compilation of previously published α-parameters and shows the affinity of binding to various inorganic cations The obtained values are highly depending on method of preparation of liposomes and on the nucleic acid type and concentration. Our presented results are obtained with preferred and reliable extrusion method of ULV preparation, creating a homogeneous vesicle suspension.
The observed size-dependent lipid-DNA interactions are in agreement with previous studies on certain plasmids and calf thymus DNA31. Thus, the kinetics of aggregation of larger nucleic acid with vesicles is slower than in the case of smaller polynucleotides. However, in addition to this data confirmation, our current study showed that the complexation is dependent on polynucleotide chain conformation.
With regard to metal cation specificity, comparison with Table 3 shows the same behaviour of Mg2+ and Ca2+, irrelevant of preparative details. The effect of Ca2+ is comparable to that of Mg2+. The slightly more efficient capacity of ternary complex formation of Ca2+, compared to Mg2+, is due to variations arising from different ionic radius effects.
Apparently, hydrophobic and electrostatic interactions, as well as the structural amphiphilicity of the bilayers govern the polynucleotide-liposome self-associations.
The values obtained for α parameter are in good correlation with binding constants of divalent metal cations with phosphatidylcholines, the affinity of binding to which decreases in the order: Mn2+ > Mg2+ > Ca2+ > Co2+ > Ni2+ > Sr2+ > Ba2+27. In comparison, the preferred nucleic acid interaction with metals via phosphate oxygen atoms indicate major thermodynamic metal on coordination sites. Thus, the affinity for base relative to phosphate increases in the order: Li+ > Na+ > K+ > Rb+ > Cs+ > Mg2+ > Ca2+ > Sr2+ > Ba2+ > trivalent lanthanides30. Raman spectroscopic investigation of calf thymus DNA, employed also herein, provide evidence for the following order of ionic perturbations on nucleic acid duplex: DNA < Sr2+ < Ba2+ < Mg2+ < Ca2+ < Mn2+ < Ni2+ < Cd2+ 32.
Hence, there is obvious temperature-dependent difference between alkaline earth- and transition metal-DNA complexes. Since, the observed trend is a function of double- or single-stranded DNA, polynucleotides with these conformations were examined in the current study. Interestingly, regarding the affinity for complex formation with neutral lipids and polynucleotides Mg2+ and Ca2+ are intermediate between other alkaline earth and transition metal associations.
The ternary complex was destroyed by treatment with 10 mM EDTA, in agreement with V. V. Kuvitchkin and A. G. Sukhomudrenko (1987)27. Apparently, the divalent cations act as salt linkages between phosphate moeities of lipids and poly(ribo)nucleotides and form the ternary complex poly(ribo)nucleotide-divalent metal cation-phospholipid vesicles.
The presented turbidimetric data (Fig.2, Tables 1 and 2) showed that interaction of DNA with lipid vesicles in the presence of divalent metal cations is characterized by increased turbidity of the system, resulting from the liposome aggregations and fusions33. Thus, decrease in turbidity at lower temperatures (approx. 37º C) is probably due to phase transition of small vesicles and a decrease at about 44 º C with larger vesicles or multilayer.
An increase of turbidity between 38 º C and 43 º C is attributed to the fusion of small vesicles. The turbidity changes were interpreted for various modes of vesicle preparation to confirm the reproducibility of the turbidimetric measurements.
This is essentially temperature-dependent process, as proved originally by E. E. Bichenkov et al. (1988)34, creating difficulties in UV/VIS registration of DNA-melting pattern. Microcalorimetric measurements were performed to distinguish between conformation-dependent modes of complexation. Fig.3 depicts DSC heating scans of binary complexes between poly(ribo)nucleotides and calf thymus DNA with DPPC-MLV in the presence of Mg2+. Mg2+-ions affects slightly the apparent specific heat capacity (Δ Cp) (Fig.3 (1) vs. (3) and (4)).
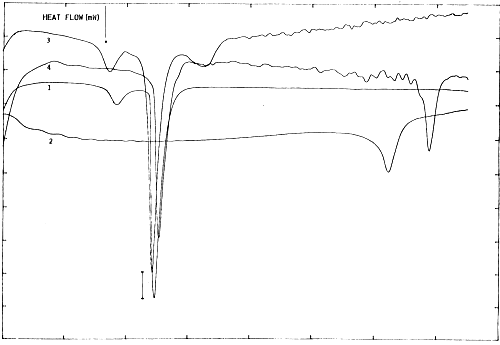
Fig. 3. Microcalorimetric phase behaviour of DPPC multilayers in the presence of poly(ribo)nucleotides and Mg2+. ΔCp bar is 0.5 J.K-1. Concentration of the synthetic biopolymers were: 1 mM of (A):(U) bp of poly(A:U) duplex (Sigma), and 1 mM of DPPC (assuming average molecular mass of dimensionless 770) and DNA (assuming average molecular mass of base pair of 643 and molecular weight of calf thymus DNA of 1,800 kDa) in 10 mM HEPES/10 mM NaCl; pH=7.2, cell volume 1.5 ml. Differential scanning microcalorimetric measurements were performed using SETARAM DSC microcalorimeter, equiped with Hewlett-Packard PC and with company supplied computer programme. (1): DPPC-MLV+MgCl2; (2): poly(A:U)+MgCl2; (3): DPPC-MLV+poly(A:U)+MgCl2; (4): DPPC-MLV+calf thymus DNA.
Interestingly, the phase separation between DPPC-MLV and poly(A:U) (Fig.3(3)) indicates that interaction and stabilization of polynucleotide duplex occur even in the absence of bridging metal cations. This result is in agreement with Bichenkov et al. (1988)34.
The effect of polynucleotide chain length and conformation is more apparent. Thus, the apparent specific heat capacity is higher for poly(A:U)n compared to calf thymus DNA, indicating that nucleic acid melting behaviour is somewhat dependent on base composition (homopolymer vs heteroduplex) and sequence19.
These ΔCp values were further used for comparisons of the transition enthalpies, ΔH, of different duplexes, which should be done for a common reference temperature, Tref. This yields duplex transition enthalpies at Tref, ΔHTref, depending much less on duplex conformation, base composition or sequence19. Although close in magnitudes, the values ΔCp were different for various duplexes and are functions of temperature and salt concentration. Such changes in adiabatic compressibility in biopolymer transitions can be expressed as the sum of intrinsic and hydration contributions19.
Fig.4 indicates that Tm value of DPPC-MLV increases upon ternary complex formation with calf thymus DNA driven by Mg2+ from 41.68ºC to 44.24ºC. In order to unravel further details of ternary complex formation, the above mentioned melting behaviour of zwitterionic lipids in the presence of DNA and Mg2+ was followed with ULV. Adiabatic differential scanning microcalorimetry (DASM-4) was used solely for comparative purposes in terms of instrumental drift (Fig.3 and Fig.4).
Since, the peaks are observed closely to each other, only results with major transitions are shown for convenience.
Fig.4. DSC heating scans of the main phase transition of DPPC multilayers and unilamellar vesicles obtained from them, upon their associations with various amounts of calf thymus DNA and 0.5 mM Mg2+. Each complex is denoted in the abbreviated form. Data is presented as excess apparent heat capacity (DCp) vs. temperature (T°C) curves. The thermograms are separated from each other at suitable distances for better representation. Details of sample preparation and measurements are outlined in Materials and Methods.
Fig.4 represents typical thermograms of DPPC liposomes and their ternary associations with calf thymus DNA and Mg2+. The first curve at the top is a calibration mark and shows a characteristic DPPC multilayer phase transitions, with pre-transition temperature peak at around 36 º C with ΔHcal=3.9 kJ/mol and the gel-to-liquid crystal, or main-phase transition temperature at 41.9 º C, in good agreement with the relevant database (LIPIDAT): http://www.lipidat.chemistry.ohio-state.edu. Next curve is the change of lipid phase transitions after complex formation with DNA in the presence of Mg2+.
DPPC-ULV’s thermogram peak appears broader with a decreased maximum. The pre-transition disappears. This melting behaviour indicates that phase separation occurs between lipids, nucleic acid alone, and their complexes. Thus after, the first peak corresponding to DPPC-ULV at 41.9 º C, a second peak appears at 51.3 º C for the equimolar mixtures of lipids and DNA. A third minor peak corresponding to free and unbound DNA alone is seen at ~60 º C.
The observed peak distribution indicates that the fraction of liposome-free DNA is much less than that in the complex. The liposome-DNA association results in the significant decrease of the ΔCp. The third curve is a phase behaviour ULV-Mg2+-DNA at 1:1:1 molar ratio. Two peaks seen correspond to lipid phase and to lipid-DNA aggregate, respectively. The latter peak is attributed to the high temperature stabilization of the DNA secondary structure, by a tight packing of DNA molecules with several ULVs, bridged by Mg2+ (Fig. 4).
The observed association is governed by surface cationization of the vesicles, sensed by a conformational change in the choline group of DPPC. It tilts towards the bilayer surface plane since its positively charged quaternary nitrogen is attracted by the opposite charge of the nucleic acid.
The ternary complex of DNA-metal ion-phosphatidylcholine vesicle remains stable at different incubation times, in agreement with small- and wide-angle X-ray scattering measurements20. Apparently, Mg2+ decrease the DNA-effective radii and create groove narrowing. This is accomplished by ligand binding to six or eight water molecules, or alternatively, to the phosphate of the nucleic acid in the minor groove in a fully hydrated state35.
The last curve at the bottom represents the equimolar mixture of DNA and Mg2+ and DNA, which brings about a major signal at ~90 º C. The Mg2+-ions at the equimolar amounts with DNA increases the Tm value by 33.7 º C, due to Mg2+-induced duplex stabilization. ULVs treated with the same Mg2+ concentration did not produce such a shift, which is normally detected spectroscopically (Fig. 4).
The figure depicts the effect of inorganic cations on phase transitions. Such binary polyelectrolyte associations comprising the interest in metal ion-phospholipid complexes has arisen from studies on ion fluxes through cell membranes and their quantitative descriptions with link to relevant functional implications. Most of the biomembranes are bilayers in appearance. Ions affect aggregation properties of the amphiphiles and thus their phase transitions36. Since their surroundings are salty biological fluids, laboratory data obtained for inorganic ion effects on artificial lipid bilayers can be extrapolated to biological membranes.
Besides their well-documented physicochemical parameters, biologically active Ca2+ and Mg2+ were chosen in the present study herein for approaching the currently available electrostatic theories for self-assemblies in aqueous media37. Metal ion binding to oppositely charged amphiphiles is well-documented. On the other hand, cation binding to zwitterionic lipids is less known. Currently, difficulties exist in interpretations of the estimated stoichiometry of cation-phospholipid interactions, as well as issues concerning the determinations of their binding constants and binding energies36. Therefore, our experimental design could give further evidence for the mechanism of metal ion-mediated supramolecular self-assembly formations.
The relevant signal indicating ion effect on lipid phase transitions was not seen. Such effect is usually reported by other authors18,38. As oppose to this results, the turbidity measurements indicated depicted this effect more clearly (Fig.5). The observed discrepancy indicates the need for coupling the calorimetric free energy estimates with one or more spectroscopic or microscopy techniques.
Fig.5. The effect of Ca2+ and Mg2+ on phase transitions of DPPC multilamellar dispersions. The main phase transition temperature of the lipids is shifted towards higher values. Thermotropic phase behaviour of lipids is presented as measurements of the change in optical density at the constant rate (2±0.2˚C) as a function of temperature. Values were recored by stepwise increasing the temperature using 3 ml quartz cuvettes thermostatized within ±0.3˚C by circulating water bath connected to the cuvette holder, as described in Materials and Methods. Recordings were performed after maintenance of the sample in the cuvette holder for 5 minutes at each temperature. Data is presented as a mean±S.D. of 6 measurements, and plotted using MATLAB® software.
More specifically, in our opinion, the use of isothermal titration calorimetry and surface plasmon resonance is a reasonable option. Since addition of EDTA diminishes the aggregation parameter of polynucleotide-divalent metal cation-DPPC mixtures in both DPPC MLV and ULV cases, obviously Ca2+ and Mg2+ affect the structure of water bathing the lipid aggregates. This is a function of the buffer and ionic strength conditions employed. Therefore, hydrophobic interactions become important, since addition of divalent cation changes the structure of the water. Such bound water exerts effects on the hydrophobic term of the free energy of the lipid aggregation and thus the lipid phase transitions as well.
When limited hydration is used, such inorganic cations influence osmotically the lipid aggregates, by competing with them for water of hydration. Hence, the effective number of water molecules available for amphiphile hydration is reduced. Thus, the osmotically driven lipid dehydration influences the lipids by increasing their phase transition temperature. Compared with this indirect effect of metal cations on water structure and their distributions around the polyelectrolites engagad in self-assembly formation, binding of these ions to charged phospholipids act by inducing lipid crystallizations, which raises the lipid Tm36.
In this context, experimental efforts have been devoted to the mode of binding and its effects on order-disorder temperature shifts of phosphatidylcholines. Neutron diffraction study revealed the particular effect of Ca2+ on structure of DPPC membranes in both solid and fluid lamellar phases, in terms of Derjaguin-Landau-Verwey-Overbeek (DLVO) theory39.
Apparently, temperature-dependent Ca2+ adsorption on the surface of the lipids induces electrostatic repulsion, which are equilibrated by van der Waals attraction forces, as deduced from calculations of Gouy-Chapman-Stern theory using the binding parameters of to DPPC. FTIR analysis showed hydrated vs dehydrated media effects upon metal cation binding to ap rotamers of a4 of DPPC choline group40. The described conformational effect on the choline head group is in agreement with 2H-NMR measurements41.
The reported scan at Fig.4 is a reproducible measurement of Fig.3, indicating a lack of instrumental drifts. The results were confirmed in Tris-HCL, HEPES and SSC buffers (data not shown). Obviously, divalent metal cations did not contribute to the stabilization of the ternary complex towards higher temperatures. While the latter effect is attributed in this case to polynucleotide chain, apparently Mg2+ creates polymorphic phase transitions in the phospholipid moiety.
The metal ion binding properties are similar for both low molecular weight polyribonucleotides (Fig.3) and high molecular weight calf thymus DNA (Fig.4). Thus, in both cases close stability constants have been observed. This is considered as indication that several divalent metal cations are bound with outer sphere coordination. Depending on particular type of the cation, this model considers a water molecule between the metal ion and the phosphate oxygens.
Extending further this case from polynucleotides to larger nucleic acids requires referring to Mg2+ hard or class a metal ion properties. Among biologically relevant divalent cations, the hardness of Mg2+ (32.55 eV) is lower than the value for the hardest metal Be2+ (67.84 eV), but superior to Ca2+ (19.52 eV), Zn2+ (10.88 eV), Cu2+ (8.27 eV) and Fe2+ (7.24 eV)42.
In an attempt to understand the cation-water interaction at the first and second level of hydration, and carrying the ab initio Hartree Fock, Density Functional Theory (DFT) and reaction field methods, the latter study indicates that a magnesium crystallohydrate in complex with 6 water molecules possess a significant difference in relation with intermolecular movements, as seen from its vibrational spectra.
The entropy contributions could stabilize this hexahydrated form of Mg2+. Within this respect Mg2+ prefer to bind to the hard oxygen sites of the phosphate groups of nucleic acids. This phosphate-binding stability is favoured by the decrease in solvent polarity. In this property, Mg2+ is more efficient compared with monovalent ions.
This seems to be due both to the counterion condensation and residual ion atmosphere screening for divalent relative to univalent cations, as well as to the Mg2+ ions complexation to phosphates in ds DNA30. Mg2+ raises the structural stability of the nucleic acid by affecting the helix-coil transition temperature of the duplex, due to partial neutralization of charges of phosphate groups with each other. The purine N7 positions are the most nucleophilic and form the preferred negative patches for ion binding, reflecting the trend for coordination with the softer donor ligand. The effect is governed by both thermodynamic and kinetic factors43.
In general, Ca2+ and Mg2+ affect order-disorder properties of DPPC in a similar way, i.e., the stability of complexes formed increases with increasing ligand charge. Usually obtained small enthalpies indicate mainly entropy driven associations. Despite the general belief for similar action of these ions, Mg2+ was seen to be much more effective in aggregation of the ternary complexes presented in the current study. This effect is explained in terms of the current view on Mg2+ vs Ca2+ chemistries.
The above mentioned ionic radius reasoning (Mg2+, 0.6 Ǻ; Ca2+ 0.95 Ǻ) governs the differences in structure, ligand binding affinity, and reaction kinetics18. The kinetic differences presented for these two ions, are due to packing effects rather than due to total bond energy. In terms of octahedral surrounding of donors of oxygen atoms, this implies that bond distance of Mg-O being close to 2.05±0.05Å, is in contrast with the irregular coordination geometry, bond angle and distance, as well as coordination number for the larger Ca2+ ions. The Mg2+ octahedron is not easily broken. Thus, the central field of Mg2+ dominates the coordination sphere.
With regard to crowding effects, the stability constants for Ca2+ exceeds those for Mg2+. This forms the basis of the differences in complex formation of these two ions. Hence, steric and pKa factors determine the strong associations of Mg2+ and in the case of its complexes with nucleic acids, much of its coordination sphere is made up from water molecules18,44. Mg2+ has more profound tendency for binding to nitrogen donors, as oppose to Ca2+.
The slow exclusion of Mg2+ permits its dominating role over Ca2+ in charge neutralizations in cellular cytoplasm. In this respect, using differences in size, biosystems remove more easily Ca2+ than Mg2+ from the cytoplasm, indicating the prevailing role of the latter in polyelectrolyte complexations.
The structure of the interacting biopolymers in lipoplex formulations is a matter of controversy. Research evidence indicates that both DNA45 and lipids46 affects each other’s structural transitions during complex formation. Most of the interpretations for this self-assembly in terms of well-established polyelectrolyte theories for interactions between oppositely charged macromolecules is an oversimplified view of the real structures.
It is likely that structures similar to cationic lipid-DNA complexes are formed47,48. In our opinion, kinetic vs. thermodynamic stability features govern this self-association in more complex way. Within this respect, the model considers the lipid-DNA structures as overlaying layers of DNA adsorbed onto lipid bilayers, after charge neutralization (Fig.6).
Fig. 6. Divalent metal cation bridged zwitterionic multilamellar (a) and unilamellar (b) vesicle-nucleic acid aggregation and fusion. Me2+ refers to inorganic divalent metal cations.
The process is governed by adsorbed cations (Me2+) on the surface of the zwitterionic lipids. The presence of such multilayers of alternating lipid-DNA assemblies is due to formation of condensed DNA as parallel arrangements between the lipid bilayers49. This is expected, due to the existence of 3-D correlation forces between the DNA-covered lipid layers, following DNA-driven formation of multilamellar liposomes from ULVs.
Thermodynamic measurements reported in Fig.3 and 4, represent probably several intermediary structures50. In this self-assembly, first the electrostatically controlled formation of lipid-DNA structures is expected. Metal cation-induced DNA condensation and compaction attracts liposome tetramers. In this structure, the accumulated positive charge densities of the liposomes would probably cause the closely situated liposomes to repel each other and dissociate from the nucleic acid. This represents a case of complexation between DNA with several MLVs assembled as grapes around Mg2+-induced compacted, branched DNA molecules (Fig.6a).
The case with ULVs is similar, but the nucleic acid helix is shown as a linear chain (Fig.6b) for representation purposes-for better appreciation of DNA-induced liposome fusion leading to generation of liposome, with higher curvature, which is advantageous for further increasing the transfection efficiency.
Another possibility could be a lipoplex, in which DNA is encapsulated within the liposome. This model considers the fact that divalent cations could be evenly distributed on both outer and inner bilayer leaflets. Hence, most probably, the DNA may be attached to inner membrane rather than moving free in the central aqueous core.
A third model could be DNA surrounded on both sides by lipid leaflets, thus forming a multilayers of lipid-DNA arrangements. This could happen as a result of the nucleic acid entrapment between the lipid bilayer during liposome fusion, which would bring the bilayers in close contact zone. This is a highly probable structure, which is essentially a DNA-driven process.
Alternatively, no any liposome is formed at all, but instead the DNA is coated with lipid pieces during preparation. Yet another possibility is the transient structure, in which DNA passes through the bilayer to interact with positive charges on the inner and outer leaflets of the bilayer.
In such a bilayer traversal, the DNA would be capable of acting as a fusogenic agent, by causing both aggregation and fusion of the vesicles. Since MLVs are highly resistant to nucleic acid penetration, Fig.6b comprises the most probable structure with ULVs. In our opinion, co-existance of several of these lipid-DNA self-assemblies is also possible, perhaps in a dynamic equilibrium. Since the pre-formed liposomes were used in the present study, to which DNA is added, the nucleic acid is likely to be adsorbed onto the outside of the vesicle and may act as a fusogenic agent, bringing liposomes in close proximity and causing its own encapsulation in a larger vesicle.
Whether this is the end result, or the last mixture leads to formation of MLVs, with little use in transfection depends on size and conformation of the nucleic acid, on the method of preparation of lipoplexes. It seems, however, that DNA size does not contribute to stability of lipid-DNA self-associations, but rather to efficiency of gene delivery.
Despite the lack of ideal model concerning the structure of the DNA within the lipid surroundings, a local unwinding depicted at Fig.6b is likely to occur, since a significant disruption of the planar interactions between the bases provide the direct explanation for the characteristic shifts in spectroscopic signals51.
The process is hydrophobically and electrostatically controlled, since liposomes aggregate and fuse in the presence of oppositely charged particles52. In the currently presented system, zwitterionic liposomes fuse in the presence of Mg2+. Hence, polyanions like DNA play an active role in adhesion, aggregation and fusion, by bridging two liposomes in close contact, with surface adsorbed metal cation-induced fusion. Since, a variety of possibilities exist regarding the structure of liposome-DNA formulations, it seems that, besides contributions due to charge neutralizations or relative lipid/DNA ratios, the absolute concentrations of the engaged system components play an additive role in thermodynamically preferred lipoplex structure formation.
The major problem with nucleic acid delivery employing liposomes as a therapeutic gene vector is efficient escape from the clearance by the reticuloendotelial system (RES)53,54,55,56. The immune response is due to accumulation of serum proteins on the liposome surface, resulting in rapid removal primarily by liver, spleen, lymph nodes, and lung tissue, the process being referred to as opsonization. Usually neutral multilamellar and unilamellar vesicles follow a slower clearance rate than negatively and positively charged MLVs. ULVs are characterized with longer residence time than MLVs54.
The unavoidable hurdle until now has been the final fate of liposome, which are engulfed by macrophages of the RES, via the lysosomal participation (Fig.1). Such fusion with lysosomes leads to destruction of liposomes through the action of phospholipases with subsequent release of vesicle’s content. Hence, efficient strategies to overcome opsonization of lipid vesicles have been designed, thus developing the concept of liposome targeting to specific target cells and tissues.
Initially, conjugation of various polymers and other macromolecules for this purpose was highly cell type-dependent and ended with discouraging results. Therefore, besides the required knowledge on the influence of in vivo environment on both vesicle leakage and clearance rates searching for improvement of the specificity of cell and tissue recognition becomes essential. The objective is to deduce alternative route of successful gene delivery avoiding the receptor-mediated intake. With this respect, we have worked on electrostatic control of membrane destabilization.
Liposomally entrapped genes would mimic the efficient viruses, acting via membrane fusion with the target cell surface2,53. We have focused on divalent metal cations and their ability for electrostatic stereocomplex formation with both amphiphiles and nucleic acid duplexes. Metal cations therefore are able to form numerous amphiphilic or hydrophobic regions and thus can potentially actively participate in membrane destabilization.
Fig.6 shows the simplified mechanism of the proposed DNA-induced fusion of liposome with host cell membrane. The initial structure of the entrapped DNA within liposome interior is based on Fig.6b, originally described by V. V. Kuvitchkin and A. G. Sukhomudrenko (1987)27, whereby DNA aggregates several vesicles leading to fusion of ULVs, triggered by temperature-dependent polynucleotide chain melting. Thus, a structure with larger curvature is formed, which is advantageous in further cell fusion.
The employed divalent metal cations have net positive charge, are capable of aggregation to form larger multimers, and have membrane destabilizing properties. Based on these properties of the surface active compounds under study, it is proposed that similarly to cationic peptides53, an inorganic cation-mediated cell membrane destabilization occur. This event is based mostly on overall three-dimensional arrangements of polar and hydrophobic regions, rather than on secondary structural elements53,57. Thus, the DNA-triggered and metal cation-induced destabilization with further disruption of negatively charged cell membrane is governed by electrostatic and hydrophobic forces.
As shown on Fig.6, metal cations bring the neutral liposomes with the entrapped therapeutic gene in close proximity with polyanionic cell lipid surface. Since, this is a salt concentration-dependent event, apparently this indicates the effect of screening on the negatively charged phospholipid headgroups ending with their destabilization.
When optimal combination of surface electrostatic and hydrophobic, as well as structural amphiphilicity is reached, DNA-induced fusion between zwitterionic liposome and host cell membrane takes place58 and Fig.7. The exact mechanism of DNA-exerted lipid fusion in the presence of metal cations is currently unknown. It could be suggested that, similarly to positively charged peptides53, the electrostatic screening of the inorganic cations’ hydration shell compensates for the inter-vesicle repulsive forces. Once the opposing membranes are in close contact zone, the electrostatic and hydrophobic features of metal cation form a link between liposome and cellular phospholipids with subsequent lipid mixing.
Fig. 7. Proposed mechanism for cellular internalization of divalent metal cation mediated DNA-induced zwitterionic lipoplex formulation.
This model is in agreement with original proposal of V. V. Kuvitchkin and A. G. Sukhomudrenko (1987)27 (Fig. 5). Our proposal is supported by recent data of Sato et. al.(2003) 59 on Mg2+-induced DNA attachment to phospholipids. However, more data is needed to clarify which lipid fusion intermediates are formed in the presence of melting DNA. Discussions concerning relevant implications of lipid fusion competent lipid vesicles of this sort remains speculative. One hurdle is the current uncertainty on the effect of lipid type and electrostatics in controlling fusion.
The presented work describes preliminary phase behaviour of simple synthetic phosphatidylcholines. Even though it is proposed as a possible experimental design, it seems too oversimplified for the in vivo situation. More specifically, the mechanisms by which the particularly employed lipid and its surface curvature with its various states of fluidity contributes to membrane destabilization is not understood at present54.
The encouraging item, however, is that liposomes normally could protect their nucleic acid content from enzymatic degradation, as deduced from routine EtBr electrophoretic pattern. Moreover, since this system possesses structural versatility, its transfection efficiency could be further improved by control of surface charge, size and lipid composition, besides other factors, together with plasmid vaccine54.
Neutral liposome-Mg2+-DNA formulations described herein are highly dynamic structures, thus frequently changing their pharmacological features. On the other hand, the ternary complex appears to be reproducible liposome-DNA formulation, but its serum stability is not yet well-defined. While promising results are reported recently on their employment60,more immunological experiments are needed for their in vivo evaluation.
Nevertheless, this preliminary thermodynamic study on the stability of neutral liposome-polynucleotide nanostructures should contribute further to design and characterization of novel lipoplexes with improved transfection properties and thus for the development of better gene transfer systems for use in human gene therapy.
The concept of metal-based pharmaceuticals will open new insights for approaching the question of whether these artificial metal complexes follow the same principles in binding to their cellular receptors.
Acknowledgment
I thank Prof. R. I. Zhdanov (V. N. Orekhovich Institute of Biomedical Chemistry, Russian Academy of Medical Sciences) for introducing me to the topic of nucleic acid-membrane interactions and for his help as my postgraduate supervisor. The hospitality and close supervision of Prof. P. Bálgavy (J. A. Comenius University-Bratislava) and Dr. J. Bagelova (Institute of Experimental Physics, The Slovak Academy of Sciences-Košice) is greatly acknowledged.
REFERENCES
1.- Kabanov AV, Kabanov VA. DNA complexes with polycations for the delivery of genetic material into cells. Bioconjug Chem. 1995; Jan-Feb;6(1): 7-20.
2.- Templeton NS. Developments in Liposomal Gene Delivery Systems. Expert Opin. Biol. Ther. 2001; 1: 1-4.
3.- Liu F and Huang L. Development of Non-Viral Vectors for Systemic Gene Delivery. J. Contr. Release 2002; 78: 259-266.
4.- Templeton NS. Liposomal Delivery of Nucleic Acids In Vivo. DNA Cell Biol. 2002; 12: 857-867.
5. Templeton N S. Gene and Cell Therapy-Therapeutic Mechanisms and Strategies. 2nd Ed., Marcel Dekker, 2003.
6.- Miller D. The Problem with Cationic Liposome/Micelle-Based Non-Viral Vector Systems for Gene Therapy. Curr. Med. Chem. 2003; 10: 1195-1211.
7.- Miller AD. Nonviral liposomes. Methods Mol. Med. 2004; 90: 107-38.
8.- de Gennes P-G. Problems of DNA entry into a cell. Physica A, 1999; 274: 1-7.
9.- Kootstra, NA and Verma IM. Gene therapy with viral vectors. Annu Rev Pharmacol Toxicol 2003; 43: 413-39.
10.- Gebhart CL and Kabanov AV. Evaluation of Polyplexes as Gene Transfer Agents. J. Contr. Releas., 2001; 73: 401-416.
11.- Anwer KB, Rhee G and Mendiratta SK. Recent Progress in Polymeric Gene Delivery Systems. Crit. Rev. Ther. Drug Carrier. Syst. 2003; 20(4): 249-293.
12.- Dass R. Cytotoxicity Issues Pertinent to Lipoplex-Mediated Gene Therapy In-Vivo. J. Pharm. Pharmacol. 2002; 54: 593-601.
13.- Ulrich AS. Biophysical Aspects of Using Liposomes as Delivery Vehicles. Bioscience Rep. 2002; 22, 2: 129-149.
14.- Vlassov VV, Balakireva LA and Yakubov LA. Transport of oligonucleotides across natural and model membranes, Biochim Biophys Acta, 1994; 1197: 95-108.
15.- Lambert G, Fattal E, and Couvreur P. Nanoparticulate systems for the delivery of antisence oligonucleotides. Adv. Drug Del. Rev. 2001; 47: 99-112.
16.- Kharakoz DP, Khusainova RS, Gorelov AV, and Dawson KA. Stoichiometry of Dipalmytoilphosphatidylcholine-DNA Interaction in the Presence of Ca2+: a Temperature-Scanning Ultrasonic Study. FEBS Lett. 1999; 446: 27-29.
17.- Lam MI, Cullis PR. Calcium Enhances the Transfection Potency of Plasmid DNA-Cationic Liposome Complexes. Biochim. Biophys. Acta 2000; 1463: 279-290.
18.- Fraústo da Silva JJR and Williams RJP. The Biological Chemistry of the Elements. The Inorganic Chemistry of Life, Chapter 9: The Biological Chemistry of Magnesium:Phosphate Metabolism, 2nd. ed., Oxford University Press, Oxford, New York, 2001, pp. 251-276.
19.- Chalikian TV, Völker G, Plum E and Breslauer KJ. A more unified picture for the thermodynamics of nucleic acid duplex melting: A characterization by calorimetric and volumetric techniques. Proc. Natl. Acad. Sci. USA, 1999; 96: 7853-7858.
20.- Uhrikova D, Rapp G, Kunst BH and Balgavy P. Interaction of DNA with DPPC Bilayers in the Presence of Mg (II) Ions, 1998; EMBL DESY Outstation – Hamburg; Project No: ML-98-6.
21.- Koynova R, Koumanov A, and Tenchov B. Metastable rippled gel phase in saturated phosphatidylcholines: calorimetric and densitomatric characterization. Biochim. Biophys. Acta, 1996; 1285: 101-108.
22.- Sorokin VA, Valeyev VA, Gladchenko GO, and Blagoi Yu P. Features of the interaction of bivalent metal ions with homopolynucleotides in the multichain conformation. Biophysics, 1994; 39,5: 821-832.
23.- Süleymanoğlu E. Turbidimetric Study of Interaction Between Polyribonucleotides, Phosphatidylcholine Vesicles and Metal Ions, NATO-Advanced Study Institute Proceedings: Trafficking of Intracellular Membranes: From Molecular Sorting to Membrane Fusion, June 19-30, 1994; Espinho, Portugal.
24.- Chong CS and Colbow K. Light scattering and turbidity measurements on lipid vesicles. Biochim. Biophys. Acta, 1976; 436: 260-282.
25.- Matsuzaki KO, Murase K, Sugishita S, Yoneyama K, Akada M, Ueha A, Nakamura and Kobayashi S. Optical characterization of liposomes by right angle light scattering and turbidity measurement. Biochim. Biophys. Acta, 2000; 1467: 219-226.
26.- Gamon BL, Virden JW, and Berg JC. The aggregation kinetics of an electrostatically stabilized dipalmitoyl phosphatidylcholine system. J. Coll. Interf. Science, 1989; 132, 1: 125-138.
27.- Kuvitchkin VV and Sukhomudrenko AG. Interaction of natural and synthetic polynucleotides with liposomes in the presence of divalent cations. Biophysics, 1987; XXXII, 4: 628-633 (In Russian).
28.- Ivanov II. Chapter V: Calorimetric Methods of Studying Biopolymers and Membrane Systems, in: Modern Methods of Biophysical Investigations – a Practicum of Biophysics, Ed. A. B. Rubin, Vishaya Shkola, Moscow, 1988; pp 203 – 216, (In Russian).
29.- Braun CS, Kueltzo LA and Midaugh CR. Ultraviolet absorption and circular dichroism spectroscopy of nonviral gene delivery complexes, In: Methods in Molecular Medicine, vol. 65: Nonviral Vectors for Gene Therapy, M. A. Findeis (Ed.), Humana Press Inc., Totowa, NJ., 2001; pp.253-284).
30.- Bloomfield VA, Crothers DM and Tinoco I Jr. Nucleic Acids-Structures, Properties, and Functions, University Science Books, Sausalito, California, 2000.
31.- Angelova MI and Tsoneva I. Interactions of DNA with giant liposomes. Chem. Phys. Lipids, 1999; 101, 1: 123-137.
32.- Duguid JG and Bloomfield VA. Aggregation of melted DNA by divalent metal ion-mediated cross-linking. Biophys J. 1995; 69: 2642-2648.
33.- Avramovic-Zikic O, Colbow K. Turbidity changes of lipid vesicles near the phase transition temperature as an indication of fusion. Biochim Biophys Acta. 1978; Sep 11, 512(1):97-104.
34.- Bichenkov EE, Budker VG, Korobeinicheva IK, Savchenko EV, and Filimonov VV. DNA interaction with phosphatidylcholine liposomes. Melting of DNA and phase transition of the lipid membrane in the complex. Biologicheski Membrani. 1988; 5, 8: 843-851 (In Russian).
35.- Hud NV and Polak M. DNA – Cation Interactions: The Major and Minor Grooves are Flexible Ionophores. Curr Opin Struct Biol. 2001; 11: 293-301.
36.- Hauser H. Effect of inorganic cations on phase transitions. Chem. Phys. Lipids 1991; 57: 309-325.
37.- Jullien L, Cottet H, Hamelin B, and Jardy A. Thermodynamic behaviour of a supramolecular system self-assembled by electrostatic interaction in aqueous solution. Results and theoretical analysis. J. Phys. Chem. B, 1999; 103: 10866-10875.
38.- Castelli F and Raciti G. A calorimetric study of the influence of divalent cations on the thermotropic behaviour of some phosphatidylcholines. Thermochim. Acta 1991; 186: 205-215.
39.- Tatulian SA, Gordeliy VI, Sokolova AE and Syrykh AG. A neutron diffraction study of the ions on phospholipid membrane interactions. Biochim. Biophys. Acta, 1991; 1070: 143-151.
40.- Grdadolnik J and Hadzi D. Conformational effects of metal salt binding to the polar head of phosphatidylcholines investigated by FTIR spectroscopy. Chem. Phys. Lipids, 1993; 65: 121-132.
41.- Macdonald PM, Leisen J, and Marassi FM. Responce of phosphatidylcholine in the gel and liquid-crystalline states to membrane surface charges. Biochemistry 1991; 30: 3558-3566.
42.- Adrian-Scotto M, Vasileva D, Mallet G, and Vasilescu D. About hydration of Mg2+: a quantum DFT study. Internet Electron. J. Mol. Des. 2003; 1: 1-13.
43.- Saenger W. Principles of Nucleic Acid Structure, Springer-Verlag, Berlin, Heidelberg, MIR Publishers, Moscow, 1987; (The Russian Translation).
44.- Ahmad R, Arakawa H, and Tajmir-Riahi HA. A comparative study of DNA complexation with Mg(II) and Ca(II) in aqueous solution: Major and minor grooves bindings. Biophys. J. 2003; 84: 2460-2466.
45.- Tarahovsky YS, Khusainova RS, Gorelov AV, Nikolaeva TI, Deev AA, Dawson AK, and Ivanitsky GR. DNA initiates polymorphic structural transitions in lecithin. FEBS Lett. 1996; 390: 133-136.
46.- Akao T, Fukumoto T, Ihara H, and Ito A. Conformational change in DNA induced by cationic bilayer membranes. FEBS Lett. 1996; 391 (1-2): 215-8.
47.- Lobo BA, Rogers SA, Wiethoff CM, Choosakoonkriang S, Bogdanovich-Knipp S, and Middaugh CR. Characterization of Cationic Vector-Based Gene Delivery Vehicles Using Isothermal Titration and Differential Scanning Calorimetry, in: Methods in Molecular Medicine, Vol. 65, Nonviral Vectors for Gene Therapy, Eds. M. A. Findeis, Humana Press Inc., Totowa, New Jersey, USA, 2001; pp 319-348.
48.- Safinya CR, Structures of lipid-DNA complexes: supramolecular assembly and gene delivery. Curr. Opin. Struct. Biol. 2001; Aug. 11 (4): 440-8.
49.- Gelbart WM, Bruinsma RF, Pincus PA, and Parsegian VA. DNA-inspired electrostatics. Physics Today, 2000; Sep.: 38-44.
50.- Smith JG, Walzem RL, and German GB. Liposomes as agents of DNA transfer. Biochim. Biophys. Acta, 1993; 1154: 327-340.
52.- Mel'nikov SM, Dias R, Mel'nikova YS, Marques EF, Miguel MG, and Lindman B. DNA conformational dynamics in the presence of catanionic mixtures. FEBS Lett. 1999; 453: 113-118.
53.- Fujii G. To fuse or not to fuse: the effects of electrostatic interactions, hydrophobic forces, and structural amphiphilicity on protein-mediated membrane destabilization. Adv Drug Deliv Rev 1999; 20; 38(3):257-277.
54.- Kirby CJ and Gregoriadis G. Liposomes, in: E. Mathiowitz (Ed.), Encyclopedia of Controlled Drug Delivery, Vol. 1, John Wiley &Sons, Inc., 1999; pp. 461-492.
55.- Opanasopit P, Nishikawa M, Hashida M. Factors affecting drug and gene delivery: effects of interaction with blood components. Crit Rev Ther Drug Carrier Syst. 2002;19(3):191-233.
56.- Kamiya H, Akita H, Harashima H. Pharmacokinetic and pharmacodynamic considerations in gene therapy. Drug Discov Today. 2003; 1; 8(21):990-6.
57.- Lin AJ, Slack NL, Ahmad A, George CX, and Samuel CE. Three-dimensional imaging of lipid gene-carriers: membrane charge density controls universal transfection behaviour in lamellar cationic liposome-DNA complexes. Biophys. J. 2003; 84: 3307-3316.
58.- Mönkkönen J and Urtti A. Lipid fusion in oligonucleotide and gene delivery with cationic lipids. Adv. Drug Deliv. Rev., 1998; 34: 37-49.
59.- Sato Y, Nomura S-I, and Yoshikawa K. Enhanced uptake of giant DNA in cell-sized liposomes, Chem, Phys. Lett., 2003; 380: 279-285.
60.- Esponda P, Goldstein M, and Witkin SS. In vitro transfection of the human vas deferens using DNA-liposome and DNA-neutral lipid complexes. Fertility and Sterility 2004; 81;1: 171-175.
Comment reviewer Prof. Ernesto Moro, MD. PhD. Prof. of Histopathology. Health of Sciences Faculty, University Rey Juan Carlos. Madrid. Spain.
In this work E. Süleymanoglu deals with new ways to create suitable non-viral gene therapeutic vehicles. So, he supportes the idea that DNA-lipid (lipoplexes) mixtures could improve biovailability and decrease inmmunotoxicity. The author focuses on the kinetics and thermodinamic parameters of phospholipid-nucleic acid array formations in presence of Mg 2+ emphasizing the use of zwitterionic species as an alternative to the cytotoxic cations lipids, as also was suggested by Kharakoz D.P et al (1999).
He gives us in his methods a precise and complete explanation of how he makes the liposome-nucleic acid mixtures with their UV/VIS spectrophotometry, turbidimetric and differencital scanning calorimetry analyses.
As a pathologist, I miss a morfological study in order to know the size and other measurments of that lipoplexes. Being agree it is not completely needed, that could help to understand if the vesicles proposed could interact with such a complex and most of the times impenetrable plasmalema.
Comment Reviewer Prof. Pilar Muñiz Rodríguez PhD. Biochemistry and Molecular Biology. University of Burgos. Spain.
One of the fields of study in which refers to genes transfer techniques, are those studies carried out with no-viral vector as the liposomes, with the purpose to avoid the problems derived from the utilization of virus in the transfer of genes. Currently, many works in this field are centred in the study of the stability of the complexes formed by nucleic acids and liposomes, by their possible influence in the capacity of gene transfer.
In this article, the author used the DSC (Differential Scanning Calorimetry) technique presents a study of the thermodynamic stability of ternary complex DNA-DPPC- Mg+2. Results presented show that the triple complex it remains stable at different incubation times and the DNA contribute to the stabilization of this ternary complex. These new data present a new information about of genic transport based on direct fusion with the cell membrane.
Comment Reviewer Jose Nazario PhD, . (Biochemistry). Researcher, Arbor Networks. Ann Arbor. USA.
In this article, Suleymanglu introduces new measurements into the field of DNA-liposome stability via cations. Using DSC measurements and an analysis firmly grounded in previous literature, the groundwork for additional insights into vesicle-mediated DNA delivery in vivo has been laid. The experiments are clear and direct, the results are presented with a reasonable interpretation, and the conclusions are sound.
Overall i'm much more pleased with this version of the paper. The
authors present more complete experimental results than they did in the first draft, and they present them in a manner that supports many of their conclusions more strongly.
Received: November 4, 2003.
Received corrected : March 2, 2004
Published: June 13, 2004