THE USE OF INFRARED SPECTROSCOPY FOR FOLLOWING
DRUG-MEMBRANE INTERACTIONS: PROBING PACLITAXEL (TAXOL)-CELL PHOSPHOLIPID SURFACE RECOGNITION
Erhan Süleymanoglu
Department of Pharmaceutical Chemistry, Faculty of Pharmacy, Gazi University,
Ankara, Turkey
Rev Electron Biomed / Electron J Biomed 2009;3:19-35
Comment of the reviewer Amélie Rebillard PhD. Centre de Lutte contre le Cancer Paul Papin. Centre de Recherche Inserm Nantes-Angers. Angers. France.
Comment of the reviewer Prof. Ahmad Safavy PhD. Associate Professor. Department of Radiation Oncology and the Comprehensive Cancer Center. University of Alabama at Birmingham. Birmingham, AL. USA
SUMMARY:
Over the past two decades, of the several new chemotherapeutic agents, taxol (Paclitaxel) has played a crucial role in the treatment of various malignancies, including those of the ovary, breast, lung, head and neck, esophagus, as well as Kaposi's sarcoma. Despite its well documented mechanisms of action causing cell cycle arrest and apoptosis following microtubule stabilization, further details still remain to be clarified. Alterations of lipid membrane composition of cancer cells as compared with normal cells are well established. In addition, the mechanisms of drug resistance, which severely limit the effectiveness of cancer chemotherapy are undertaken by membrane located proteins such as multidrug resistance (MDR-1) or P-glycoprotein (Pgp).
On the other hand, recent chemotherapeutic regimens employ anticancer drug induced apoptosis, during which dynamic structural changes occur in cellular dynamics characterizing cell death phase leading to fragmentation into membrane-bound apoptotic bodies. Thus, cell membranes represent an attractive research field in cellular carcinogenesis and cancer therapy. Besides DNA, plasma membrane is considered as the most important target for many antineoplastic drugs. However, its role in chemotherapy-induced cell death is not well understood. Hence, it is interesting to study the molecular interactions of the anticancer drugs in phospholipid environment. Both cell biological and biotechnological aspects are aimed.
By further clarifying the precise mechanisms of taxol-lipid interactions, better understanding of its pharmacological targets can be obtained. Moreover, gaining further insights on such drug-lipid interactions would encourage the design of novel lipid based antitumor drug formulations with improved bioavailability properties and decreased toxic side effects. The currently employed analytical approaches to follow drug-cell or drug-membrane interactions are limited by either the requirement of experienced personnel, sophisticated instruments or methodological difficulties. Therefore, developing novel experimental procedures using complementary, easier to apply, user-friendly techniques is to be emphasized.
Vibrational spectroscopy has been regarded as a suitable technique for studying drug-phospholipid complex formations. Here we report the use of infrared (IR) spectroscopy to determine dynamic structural transitions of Taxol-phospholipid binary complex upon recognition of individual molecules engaged. Spectra of unbound components were compared with those of drug-lipid binary mixtures. Data obtained for carbonyl, phosphate, choline and CH groups are used for deductions of drug-lipid recognition profiles. The observed effects are considered as asymmetric and symmetric methylene stretching frequencies, splitting of its scissoring band and broadening of carbonyl stretching mode. The possible structure of the taxol-lipid complex formation and its significance in terms of its further potential to be used as an improved drug delivery formulation is also discussed.
KEY WORDS: Anticancer agents. Taxol-lipid recognition. IR spectroscopy. Drug-cell interactions. Mechanisms of action. Drug delivery.
RESUMEN: En las dos últimas décadas, de entre varios nuevos agentes quimioterapéuticos, el taxol (paclitaxel) ha desempeñado un papel crucial en el tratamiento de varias neoplasias, entre las que se incluyen tumores de ovario, mama, pulmón, cabeza y cuello, esófago, así como el sarcoma de Kaposi. A pesar de su bien documentado mecanismo de acción, causante de la detención del ciclo celular y apoptosis tras la estabilización de los microtúbulos, quedan aun detalles pendientes de aclarar. Las alteraciones de la composición lipídica de las membranas de las células neoplásicas, en comparación con las células normales, son bien conocidas. Además hay mecanismos de resistencia a los medicamentos, que limitan notablemente la eficacia de la quimioterapia antineoplásica, debidos a proteínas de membrana, tales como la Multidrug Resistance-1 (MDR-1) o la P-glicoproteína (Pgp).
Por otra parte, regímenes de quimioterapia recientes utilizan citostáticos que indicen apoptosis durante la cual, se producen cambios estructurales en la dinámica celular que caracterizan la fase de muerte celular que conduce a la formación de cuerpos apoptóticos unidos a membrana. Por tanto, la membrana celulare representa un atractivo campo de la investigación celular en la carcinogénesis y terapia antineplásica. Además del ADN, la membrana plasmática es considerada como el objetivo más importante de muchos fármacos citostáticos. Sin embargo, su papel en la muerte celular inducida por la quimioterapia no está bien conocida. Por eso es interesante estudiar las interacciones moleculares de los citostáticos en un entorno fosfolipídico. El objetivo son los aspectos biológicos celulares y biotecnológicos.
Para aclarar los mecanismos precisos de las interacciones de taxol con lípidos, se logró un mejor entendimiento de sus dianas farmacológicas. Por otra parte, la mejor comprensión de tales interacciones de drogas y lípidos fomentará el diseño de nuevos medicamentos basados en la interacción de lípidos y citostáticos con mejor biodisponibilidad y disminución de toxicidad secundaria. Los enfoques analíticos empleados actualmente para el seguimiento de las interacciones de drogas y células y de fármacos y membrana son limitados, bien por la necesidad de personal con experiencia, bien por la necesidad de instrumentos sofisticados o por dificultades metodológicas. Por tanto, debe ser estimulado el desarrollo de nuevos procedimientos experimentales que utilicen técnicas complementarias, más fáciles de aplicar. La espectroscopia vibracional ha sido considerada como una técnica adecuada para el estudio de los complejos de drogas y fosfolípidos.
Presentamos el resultado de la utilización de espectroscopía de infrarrojos (IR) para determinar las transiciones dinámicas estructurales del complejo binario de taxol y fosfolípidos a partir del reconocimiento de moléculas individuales implicadas. El espectro de componentes no ligados se comparó con el de los complejos binarios farmaco-lipidicos. Los datos obtenidos de los grupos carbonilo, fosfato, colina y CH fueron empleados para las deducciones de reconocimiento de perfiles farmaco-lipídicos. Los efectos observados se consideran como frecuencias de vibración de metilación asimétrica y simétrica, dehiscencia de bandas y amplificación de enlaces carbonilo.
Se discute la posible estructura de la formación de los complejos taxol y lípidos y su importancia en términos de su potencial para ser utilizado como una mejora de la biodisponibilidad del fármaco.
PALABRAS CLAVE: Agentes antineoplásicos. Reconocimiento de taxol-lípidico. Espectroscopia de infrarrojos. Interacción farmaco celula. Mecanismos de acción. Disponibilidad de fármacos.
INTRODUCTION
Among the traditional cancer therapy approaches, such as surgery, radiotherapy and immunotherapy, systemic chemotherapy comprises one of the most rapidly growing fields of cancer biology and medicinal chemistry1. As cancer is considered as a signal transduction disease, oncology research currently is focused on elucidating the cellular signaling routes resulting in programmed cell death. The long-term aim is to kill malignant tumor cells by inhibiting some of the mechanisms engaged mainly in cell division2-6. As a major players of normal cell cycle events, such as mitotic spindle formation, maintanance of cell shape, organelle localization and integration of segregated DNA, microtubules comprise an attractive target for anticancer drug design. Previous studies indicate that such antimicrotubule agents are potent promoters of apoptosis in cancer cells7-9. However, their exact mechanisms of action are unclear yet. On the other hand, microtubules are considered as an important target of cytotoxic compounds of natural origin, most of which have been discovered by large-scale screening. These products are highly successful in cancer treatment and they have been suggested as the best known cancer target10. These antimicrotubule drugs act in synergistic manner and are used in combination therapies, avoiding high doses of any individual drug7-10. Being one of the major eukaryotic cytoskeletal assemblies, microtubules perform a myriad of functions including plasma membrane-associated activities11-16.
Since biomembranes themselves are considered as an important site in cancer pathogenesis17, it is interesting to study the mechanisms of action of such antimitotic drugs in membraneous surrounding. This interesting link is supported by previous reports on the role of transmembrane proteins in control of tumorigenesis and tumor progression11,17. For instance, such membrane-bound proteins activate the oncogenic receptors on cellular surfaces and thus affect indirectly microtubule dynamics and drug sensitivity11. In addition, the role of biomembranes in apoptosis and pathogenesis of disease is well established17-23. In terms of joint site of anticancer target design, studying phospholipid membrane-microtubule assemblies becomes an interesting item. Obviously, further research is to be directed towards understanding the particular roles of both cellular compartments in carcinogenesis and therapy. Thus, assorted changes in membrane dynamics that are apparent within the apoptotic cell and secretory membrane traffic arrest would be a good starting point24. In the context of such a chromosome-membrane connection, it should be emphasized that chromatin-rich surface blebs are a hallmark of the late-apoptotic cell and microtubules are actively engaged here, which is concominant with the disruption of centrosomal architecture25-27.
Further understanding of these signaling pathways could open new therapeutic opportunities for developing novel stategies for overcoming drug resistance hurdles in tumor cells1-10,29. The objective is to unravel the molecular mechanisms of cancer cell resistance to apoptotic stimuli and hence the development of novel effective therapeutic agents. The role of membrane structure and dynamics in apoptosis is exemplified by its severe disruption following cell injury17-24,28. However, the involvement of tumor plasma membrane in regulation of drug-induced effects leading to cell death is still poorly understood. On the other hand, the strong link between the phospholipid membrane fluidity and functional features of both normal and tumor cells, including programmed cell death or apoptosis, is now well established11,17. Thus, the interaction and permeability of biomembranes and the activation of cell surface receptors by ligands will be affected by the structure and fluidity of the cell membrane lipid bilayer. Most chemotherapeutic drugs and cytotoxic immune cells as well as certain microbial toxins mediate their cytotoxic action through the stimulation of apoptotic pathways leading to programmed cell death in sensitive tumor cells1-3,5,6. Resistance to apoptosis may be due to fluidity of the cell membrane. Therefore, a better understanding of the mechanisms involved in chemotherapy-induced apoptosis as a function of alterations in tumor cell membrane fluidity is essential.
Drugs interfering with cell cycle events comprise two major classes based on their effects on microtubule polymerization and the mass of microtubule polymers: those inhibiting polymerization, such as the vinca alkaloids and those stabilizing microtubules, such as the taxanes and epothilones8,10,18,30-35. The taxanes Paclitaxel (taxol) and docetaxel (Taxotere®) were the first antimicrotubule agents approved for use in solid tumors30-33. They form a group of hydrophobic compounds, approved by Food and Drug Administration (FDA) to cure breast, ovarian, non-small-cell lung and prostate cancers31. As a member of this group, taxol was discovered in 1960s within the screening program of the National Cancer Institute trying to identify novel natural compounds with anticancer properties34,35. It was initially extracted in a crude form from the bark of the North American pacific yew tree Taxus brevifolia, subsequently found to be effective against various tumors31. Unfortunately, the limited availability of the pacific yew tree bark and the poor drug solubility have been major obstacles for its clinical testing31,34-36. Therefore, the emphasis was put towards total synthesis of taxol as an alternative source37.
The physical properties of Taxol are one of the central themes in the pharmacological and pharmaceutical sciences38. Thus, its different applications have been proposed according to characteristics of its various solid states. Therefore, the control of these features becomes essential. The various ways of administration of such lipophilic drugs is often problematic due to their low aqueous solubility. Use of solubilisers and other formulations with a high dissolution rate therefore becomes a necessity in order to achieve their therapeutic dose. Phospholipids can be used to solubilise such drugs, with spontaneous formation of complexes. Thus, encapsulation of hydrophilic and binding of amphipathic as well as lipophilic drugs are possible. Studying such drug-lipid recognitions would help to obtain detailed knowledge of cellular functions of biomembranes and to build novel models for better understanding of drug action39. Factors that affect tumour sensitivity, cellular pharmacology and drug disposition are being intensely investigated trying to design taxanes with improved efficacy, bioavailability and pharmacokinetics31,32,40-44.
Pharmaceutical and physicochemical analyses of drug-membrane complexes have been studied by numerous analytical methods employing a variety of drugs and model membranes39. Various approaches have been used for structural characterization of these macromolecular associations. Because of methodological difficulties, more convenient ways are needed to control the morphologies of drugs. Vibrational spectroscopy (infrared and Raman spectroscopy) provide useful information on dynamic changes occuring after complex formation of different biomolecules45,46, and thus represents a suitable method of analysis and deserves to be employed also in drug-membane studies.
Fluorescent assays, X-ray scattering, various microscopy, thermodynamic and spectroscopic techniques employed until now in the characterization of drug-phospholipid interactions have resulted in abstract data frequently with discrepancies, which is difficult to relate to cellular mechanisms of anticancer drug action. On the other hand, despite their own drawbacks, spectrometric approaches offer definite advantages over the abovementioned methods in terms of opportunity to work with real-time, non-destructive and molecule-specific detection protocols. Among these, infrared spectroscopy (IR) and Raman scattering provide valuable information on structural changes occuring upon association of biomacromolecules45,46. IR spectroscopy and IR microspectroscopy of human cells and tissues have attracted scientific interest, since it can detect chemical composition and structural changes of cells and tissues in health and in pathology46,48. Fourier Transform Infrared (FTIR) spectroscopy has been used for studying genome damage and for developping diagnostic applications such as cancer detection and monitoring46,49.
Unfortunately, the power of vibrational spectroscopy in analysing human cells and tissues has not been appreciated yet by the biomedical researchers. These methods do not employ bulky extrinsic probes and provide with possibility to avoid peak fluctuations, as well as scattering and absorption flattering artifacts. IR spectroscopy is frequently applied for following biomolecule structure and kinetics50-52. Although, it does not provide the atomic details of X-ray crystallography and NMR spectroscopy, it is widely used, as it can distinguish between different secondary structures and motifs. In addition, little sample material is needed, spectra are collected rapidly, and the samples are used in liquid, gaseous and solid form or on surfaces 45.
The term Fourier Transform Infrared Spectroscopy (FTIR) refers to a fairly recent development in the manner in which the data is collected and converted from an interference pattern to a spectrum45-47,50,51. It is perhaps the most powerful tool for identifying types of chemical bonds45.
The wavelength of the absorbed light is characteristic of the chemical bond as shown in its annotated spectrum. Concerning FTIR spectrometer, usually, the flow cell is sandwiched between CaF2 or other plates. The IR beam from the spectrometer is focused on the flow channel of the FTIR cell by means of a parabolic mirror and subsequently on a highly sensitive detector. Bright, collimated, broad spectral IR light source is essential for time-resolved structural studies of chemically triggered biosystems. The instrument can be readily coupled to other analytical device for more precise measurements. FTIR spectra of pure compounds are generally so specific that they are considered as a molecular "fingerprint". This is acomplished by recognizing characteristic shapes and patterns within the spectrum, and by applying the information obtained from previously known group frequency data, along with other chemical and physical data from the sample45.
The aim of this study was to follow the basic features of taxol recognition with phospholipids by applying IR spectroscopic measurements. The obtained information can be used further for deductions on its precise cellular and pharmacological mechanisms of action, for improving its solubility properties by phospholipids, as well as for designing novel lipidic carriers for drug delivery.
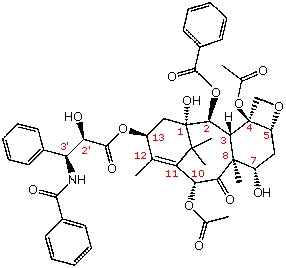
Figure 1. A structural formula of the antitumor agent taxol. The 11 chiral centers are labeled in magenta.
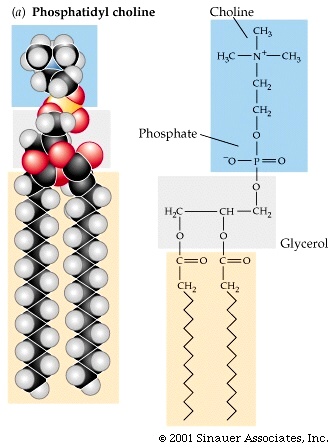
Fig. 2. The molecular structure of Phosphatidylcholine (used with permission, after
Purves et al: Life: The Science of Biology, Sixth Edition, by Sinauer Associates, Inc.).
MATERIALS AND METHODS
Materials
Egg phosphatidylcholine was purchased from Avanti Polar Lipids (Alabaster, AL, USA). FTIR-grade KBr, and Paclitaxel were a product of Sigma Chem. Co., MI (USA). All chemicals used were from the highest analytical grade.
Methods
FTIR Spectroscopy
Infrared spectra were recorded on IFS 66/S FTIR spectrometer (Bruker Analytische Messtechnik GmbH, Karlsruhe, Germany), equipped with He-Ne laser detector and KBr beam splitter. KBr pellet method was employed as FTIR sampling technique. The samples were prepared as drug/lipid mixtures in the desired ratios by using a die press. Spectra were collected after short incubation of lipid with Taxol. Interferograms were accumulated over the spectral range 4000 cm-1 to 500 cm-1, with a nominal resolution of 2 cm-1. Afterwards, the spectra of free drug and egg phosphatidylcholine were recorded. After normalization of absorbance and baseline correction, the peak frequencies of the symmetric CH2 stretching, the asymmetric P=O stretching and the asymmetric N-(CH3)3 stretching peaks were determined by using the original software, provided by the manufacturer.
RESULTS
Having considered the advantages of vibrational spectroscopy over the other analytical methods for the analysis of drug-membrane phospholipid interactions45-48, FTIR spectroscopy was applied for further characterization of molecular recognitions between Taxol and phosphatidylcholine. The final appearance of the spectrum is very important in the interpretations of drug-lipid complexation patterns. FTIR spectroscopy allows the following of acyl chain disorder and the recognition between lipids and membrane proteins expressed as the frequency of the symmetric CH2-stretching mode near 2.81 cm-1. This frequency decreases on the transition of the membrane lipids from an ordered to a disordered state. Thus low and high frequencies of the stretching mode relates to the rigid and fluid states of membrane lipids45-47,51. The popular method of compression of the drug and lipid samples with alkali metal halide pellet (KBr pellet or disk method) was applied. The procedure has its own drawbacks and difficulties concerning routine reproducibility of results and often requires a skilled operator. Otherwise, spectral artifacts and distortions can be obtained causing problems with interpretations. Furthermore, certain compounds may react with KBr, leading to either occurence of the halogen (oxidants) or halogen exchange (halide salts). Applying pressure can also change the appearance of the spectrum, especially when the sample under study exists in more than one polymorphic form60. However, when employed with these precautions the compaction of the KBr/sample mixtures did not foster aggregations, and remain conformationally unaltered61. The method is invaluable in determining the role of different functional groups engaged in such complexations39.
The samples were prepared as described in Materials and Methods. The upper spectrum denotes the reproduced pattern of free egg phosphatidylcholine. The criterion for elimination of water effect from the spectra was based on the straight baseline between 1750 cm-1 and 2200 cm-1, where the water combination mode is located. As a control, the spectrum of unbound Taxol was taken and compared with those reported in the literature previously.
IR spectral features of phosphatidylcholine
Egg phosphatidylcholine was selected for this study, due to its wide distribution in animal cell membrane leaflets and because of its well-known physicochemical characteristics (http://www.lipidat.chemistry.ohio-state.edu) both in the gel and in liquid-crystalline state. Approximately 10 well seen bands can be employed in studies of structural changes of this phospholipid following association with various ligands (Table 1 and Figure 3).
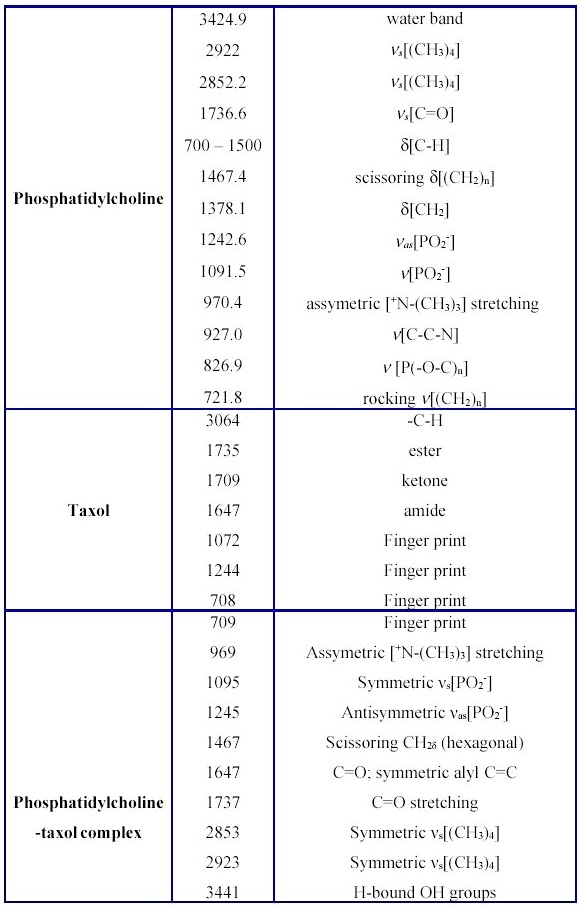
Table 1. Principal infrared absorption bands, relative intensities and frequency assignments for free Taxol, lipid and their binary complex.
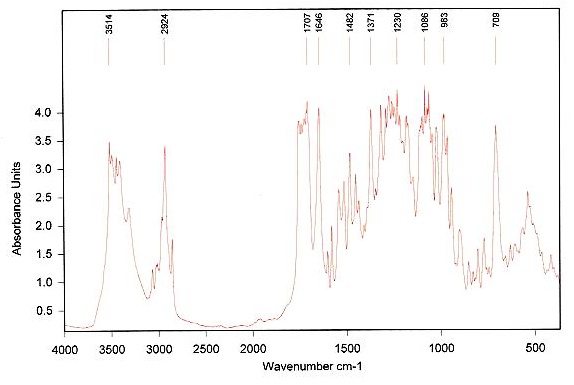
Figure 3. Infrared spectrum of taxol.
Those observed between 2750-3100 cm-1, representing CH stretching modes, those between 1150-1400 cm-1 and 1465-1415 cm-1 depicting CH2 wagging progression and CH2 scissoing vibrations are considered particularly useful62. The former is used as a measure of the degree of lipid acyl chain order, whereas the later is regarded as an indication of chain packing effects. The signal at 1258 cm-1 is ascribed to antisymmetric PO2- stretching and, that at 1736.6 cm-1-to v[C=O] stretching. The well-seen scissoring vibration at 1465-1475 cm-1 shows the existance of hexagonal packing of acyl chains. The peak at 1242 cm-1 corresponds to antisymmetric PO2- stretching and provides information about interfacial region of the phospholipid. v[C=O] stretching vibration (1736.6 cm-1) is split into two separate bands, ascribed to hydrogen unbound and to H-bonded C=O groups, respectively63. The band at 970.4 cm-1 depicts stretching of the asymetric terminal choline group. The other minor bands of egg phosphatidylcholine are located in the region 700-1100 cm-1.
IR spectrum of taxol
Under these conditions, the amorphous Taxol is converted to anhydrous state, suggesting that several solid-state structures of the drug coexist. Major lipid specific band frequencies are seen when equimolar ratios of drug-lipid complexes are used. The most important of these are those belonging to CH vibrations (2750-3100 cm-1) and CH2 wagging progression and the CH2 scissoring vibrations (1150-1400 cm-1 and 1465-1475 cm-1). These depict the conformational order and provide information on packing effects of the acyl chains, respectively. PO2- vibrations are located at 1242 cm-1. On the other hand, taxol possesses substantial bands at 1600-1750, 1180-1300 and 630-770 cm-1(Figure 4 and Table 1).
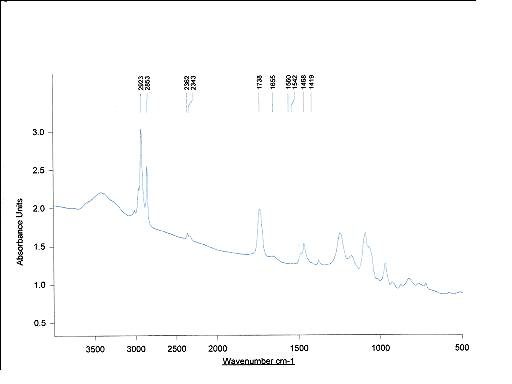
Figure 4. Complete infrared spectrum of egg phosphatidylcholine.
In comparison with the spectra of the unbound drug the lipid-bound spectral shifts of taxol's carbonyls from 1736 cm-1 to 1732 cm-1 and from 1736 cm-1 to 1707 cm-1 are seen (Figures 3-5 and Table 1). The strong bands at 3300-3500 cm-1 are due to OH-groups linked to the H-bonds.
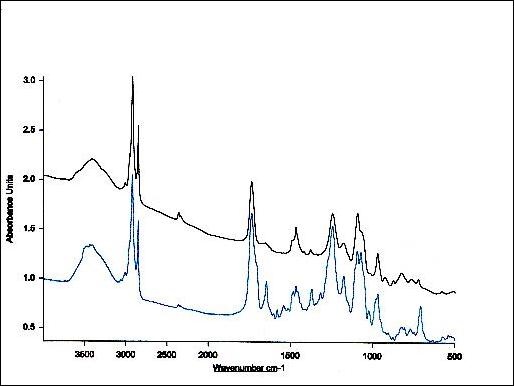
Figure 5. Infrared spectrum of equimolar mixture of taxol and phosphatidylcholine.
IR Absorption Patterns of Taxol-Lipid Interactions
Following drug-lipid recognition, the infrared absorption intensities are decreased and additional band shifts take place. This indicates a conformational restriction of lipid moiety due to bond formation with the drug, depicting the decrease in drug flexibility. In general, the overall spectrum (Figure 4) appearance provides with opportunity to perform testing for the presence of organics and hydrocarbons (3200-2700 cm-1), for hydroxy or amino groups (3650-3250 cm-1), for carbonyl compounds (1850-1650 cm-1), for unsaturation (1670-1620 cm-1), for aromatics (1615-1495 cm-1), as well as for multiple bonding (2300-1990 cm-1)60. In conclusion, FTIR spectroscopy clearly shows that Taxol-lipid recognition and binding is governed by CH and CH2 groups, as well as H-bonded surface OH-groups. In addition, PO2-, C=O, C=C and CH2 groups of the drug are also engaged. Data shows that Taxol fluidizes the upper region of the acyl chains after their experimental rehydration, whereas the hydrophobic core is rigidized. The increase in the asymmetric and symmetric methylene stretching frequencies, the splitting of methylene scissoring band and broadening of carbonyl stretching mode depict that the drug mostly recognizes the cooperativity region, binds to the surface phospholipid acyl chains and induces fluidity in their headgroup region (Figure 5).
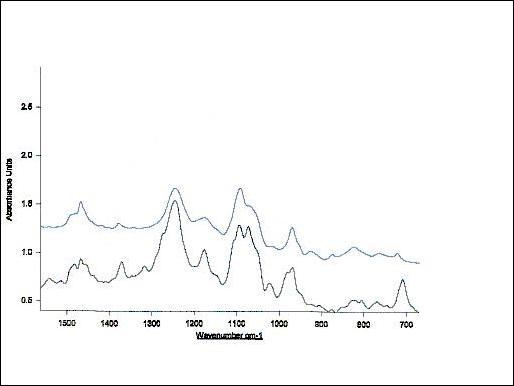
Figure 6. Infrared spectrum of equimolar mixture of Taxol and phosphatidylcholine depicting H-bonded OH, aromatic and carbonyl group frequencies (700-1500 cm-1). The upper spectra denotes unbound egg phosphatidylcholine within this spectral region.
Thus, by employing such a IR spectroscopic approach, further details of Taxol-membrane phospholipid interactions could be obtained and exploited for elucidation of the chemotherapeutic drug effects. Therefore, studying its recognition events with cellular phospholipids becomes crucial. Such biophysical and structural studies of Taxol-lipid arrangements can give valuable information about the organization of the drug in membranes of both normal and malignant cells and can help to optimize lipid matrix concerning its solubility potential.
DISCUSSION
Taxol: Mechanisms of Action, Resistance and Therapeutic Targets
Research evidence suggests that taxol kills cancer cells by induction of programmed cell death64-68. Besides mitotic phase effects in drug-treated cells, recent data indicates that taxol activates signaling molecules by transcriptional regulation of various genes suggesting that the drug initiates apoptosis via multiple mechanisms66-68. The checkpoint of mitotic spindle formation, activation of cyclin-dependent kinases (CDK), and the c-Jun N-terminal kinase/stress-activated protein kinase (JNK/SAPK) are shown to be involved in taxol-induced programmed cell death in concentration dependent manner66. The current knowledge of the molecular mechanisms of the taxol can be summarized as follows66-68:
1) the drug kills cancer cells through p53-independent pathways;
2) it kills more efficiently p53 mutated cells than those with wild-type p53 and
3) it preferentially induces G2/M arrest of the cell cycle and apoptosis in transformed cells leading to reversible G1 arrest in non-transformed cells.
Nevertheless, the cellular and biochemical events involved in taxol-induced apoptosis are not elucidated sufficiently and are a matter of intensive research, since controversial issues still remain to be clarified. This serves as a motivation to search for other cellular targets of the drug.
In general, drug resistance severely limits the efficacy of cancer chemotherapy and affects patient survival. The overexpression of multiple drug resistance (MDR-1) or heavily glycosylated 170-kDa plasma membrane protein known as P-glycoprotein (P-gp), a drug transporter belonging to the ATP-binding cassettes (ABC) superfamily of proteins is the major cause of resistance to antimitotic agents. The human ABC transporter gene superfamily comprises currently 49 members belonging to 8 subfamilies69-75. It functions as an ATP-dependent efflux transporter of multiple hydrophobic cytotoxic drugs. This single integral membrane transporter surprisingly unspecifically transports various hydrophobic peptides, cytotoxic drugs and chemosensitizers possessing different molecular structures due to their competition for a common drug pharmacophore present in P-gp74. A mechanistic basis for the basal ATPase activity involving an endogeneous lipid-soluble substrates has been proposed74,75. This suggests that peptide substrates of P-gp, particularly ionophores, which are primarily confined to the membrane, are an invaluable dissection tool for probing the intramembranal mechanism of action of the mammalian MDR.
Several mechanisms of resistance have been described for taxol. Among these are P-gp mediated drug efflux, isotype expression and altered tubulin synthesis and tubulin mutations, the latter shown by in vitro studies76. More recent proteomic analysis depicts important resistance mechanisms of the drug to be related with stress responce, metabolism, protein biosynthesis and cell cycle and apoptosis in ovian cancer cells77.
The MDR phenotype usually can be reversed by cotreatment of resistant cells with nontoxic concentrations of hydrophobic compounds known as chemosensitizers, modulators, or resistance modifiers. Among these are calcium channel blockers (such as verapamil), calmodulin antagonists and antiarrhythmia agents, antihistamines, lysosomotropic amines, immunosuppressants, steroid hormones and modified steroids, nonionic detergents, anesthetics, and various amphipatic drugs78. Since most of these P-gp mediated MDR modulators are surface-active agents affecting the lipid environment of cell membranes, studying their intermolecular interactions in the presence of relative drugs becomes essential. This would give further data on the mechanisms of MDR reversal.
Although, the use of P-gp antagonists sometimes succeeded to achieve the higher susceptibility of tumors towards chemotherapy, pharmacological reversal of MDR activity by the use of specific inhibitors of drug carriers is problematic and often leads to toxic side effects79,80. This is due to inhibition of endogeneously expressed P-gp, which has an important protective function in these tissues. An alternative approach to overcome MDR could be the use of liposomes, and more specifically of immunoliposomes, since this technology allows to by-pass drug transporters located in the plasma membranes79. Liposomes, or phospholipid vesicles, comprise an attractive research field regarding their potential to be applied as pharmaceutical carriers for anticancer drugs81. The objective is to formulate novel lipid-based drug carriers with improved bioavailability features to enhance the solubility of poorly soluble antineoplastic drugs. This would further improve the efficacy of chemotherapy and reduce their toxic side effects.
Lipid specificity and carcinogenesis
Selection of a neutral phospholipid in this study is based on the following functional data with biochemical implications. Phosphatidylcholine is involved to a significant extent in composition of lipid microdomains and rafts17,19,83-100. Recent work reports that the P-glycoproteins encoded by the MDR3 (MDR2) gene in humans and the Mdr2 gene in mice are mainly phosphatidylcholine translocators94. This is further supported by the fact that they have retained significant drug transport activity, which is suppressed by inhibitors of drug-carrying P-glycoproteins. It remains to be determined whether such translocators also transport some drugs in vivo. Recently, phosphatidylcholine was shown to be of major importance in cell division, cell signaling, malignant transformation through Ras-dependent and Ras-independent carcinogenesis99,100. Taxol recognition features of this single phospholipid were followed in the present study mainly due to its well known physicochemical characteristics, in terms of phase behaviour84 and molecular dynamics simulations98, as well as because of practical reasons, originating from studies on its liposomal incorporation. The former reasoning simplifies such a preliminary measurements and deductions drawn. At this stage, working with mixed lipid systems and incorporating cholesterol would unnecessarily complicate its physical chemistry. Liposomes containing this zwitterionic phospholipid are known to have the highest entrapment efficiency of the drug101. Therefore, the item of lipid composition and its role in carcinogenesis is interesting to study. Our measurements on specificities of taxol undertaken with positively and negatively charged lipids is in progress and will be reported separately.
Towards membrane lipid therapy
Cell membranes have always been regarded as an important subcellular organelle, both as a site of pathogenesis and as a therapeutic target17,19,20,39,54-59,83-102. Among the most popular current drug designs are those developed towards alteration of capability of mammalian cells to initiate cell suicide26,28,103. Since most of these apoptotic, as well as other normal cellular activities, take place in membraneous interfaces or surroundings, it is not surprising to suggest the design of specific therapies targeted towards modulating membrane bilayer structure and dynamics. This forms the basis of a newly emerging field of membrane lipid therapy104,105. In this respect, the interaction of various drugs with model and biomembranes is a subject of intensive research and lipid binding profiles of numerous drugs have been characterized39. More specifically, the issue of the use of lipids as targets for overcoming antineoplastic drug resistance is very interesting to study. How tumor cell membrane alterations influence the response of cancer to chemotherapy still remains to be defined. The motivation for starting research in this field comes from recent reviews on the role of lipid phase of cell membranes in P-gp-mediated MDR activity54,109,117, as well as in reversal of tumor resistance to apoptotic stimuli58.
Substantial experimental evidence shows that the growth of tumors is accompanied by alterations at the cell surfaces53-59. These changes in lipid composition, structure and function of pathobiomembranes are cell type dependent83-102. Thus, hepatomas possess decreased fluidity, i. e. increased rigidity, whereas neural tumors, lymphomas and leukemias show opposite trend56. Obviously, the capability of cancer cells to metastize appears to be related to the degree of membrane fluidity. This is achieved via signaling through plasma membrane lipid rafts involving mainly death receptor pathways59. In this respect, taxol has many effects on this membrane-linked pathway, which can briefly be summarized as follows. Taxol initiates Raf-1 activation at the plasma membrane after its recruitment by Ras110. All major mitogen-activated protein kinase (MAPK) family members, c-Jun N-terminal kinase (JNK), p38 MAPK, and extracellular signal-regulated kinase (ERK)82 were shown to be activated upon drug treatment independently of the caspase cascade111. Despite previous observations that overexpression of Akt-(protein kinase B), a Serine/Threonine protein kinase, with a pivotal role in exerting an antiapoptotic effect against various stimuli, it is yet unclear whether taxol causes apoptosis through modulation of the Akt survival pathway112. Interestingly, it was shown that certain MDR cell lines possess drug effects depending on perturbations in lipid metabolism113. Other studies also indicate membrane effects of the drug. Caveolin-1, a principal component of caveolae membranes may play a role in the development of taxol resistance in A549 cells114. Based on previous data suggesting that taxol can directly affect mitochondria perhaps through the interaction with tubulin associated with the mitochondrial membrane115 the studies on drug effects on membranes has continued and alteration of membrane asymmetry only within a narrow concentration range was found 116. Since at high concentrations, the drug exerts other effects, it was concluded that possibly taxol at high concentration may cause a variant of cell death other than apoptosis mostly associated with mitochondrial collapse. In the course of these studies, it has become clear that lipid phase of the membrane contributes somehow on taxol mechanisms in Pgp-mediated MDR events.
However, the mechanisms leading to such a chemotherapy-induced cell death remain unclarified. In malignant differentiation, the tumor cells may overcome immune surveillance by modulating and phase separating the interfacially expressed proteins55. These events are also governed by the degree of membrane fluidity changes54,58,109. Drug receptor clustering could occur at plasma membrane microdomains54. The altered membrane fluidity could also participate to the recruitment of death receptors into membrane lipid rafts upon cytotoxic taxol challenge. The fluctuations in fluidity of the existing cryptic antidramic antigens in the cancer cell membrane have opened new opportunities for curing neoplastic diseases. Autologous tumor cells with induced decrease in their lipid fluidity were shown to have an increased ability of specific immune responses, as oppose to normal control cells, subjected to the same treatment55. More specifically, since P-glycoprotein (P-gp)-the major contributor of chemotherapeutic multidrug resistance (MDR) leading to limitations in cancer treatment efficacy, interacts with its substrates after their partitioning into the phospholipid bilayer, changes in membrane physicochemical features such as fluidity alterations, may have substantial effects on its functional activity. This requires the study of drug-phospholipid interactions in more detail. In studying these cellular events, it is important to characterize the lipid targets and binding affinities, as well as lipid specificites of the anticancer drugs studied.
Exciting future directions of studying taxol-lipid interactions exist regarding novel cancer treatment strategies. Two general pathways are usually involved-the intrinsic mitochondrial and the extrinsic death receptor pathway59. The RAF proteins have a conserved role connected to the activation of the small GTPase RAS at the plasma membrane82. RAS proteins are bound to the inner surface of the plasma membane and act as a central point in cell signaling. Taxol binds directly to RAS and is recruited to the plasma membrane110-111. The majority of anticancer agents preferably induce the mitochondrial death pathway releasing compartmental pro-apoptotic molecules and activating some of the members of caspase family. These compounds can also lead to Fas death receptor aggregation at the membrane level independently of its ligand FasL, with death inducing signaling complex (DISC) formation and activation of another caspase82,103. Although research on taxol mechanisms of action focuses on microtubules and DNA31, several arguments could be raised justifying a need for searching other cellular targets. Thus, organelle or nucleic acid binding cannot explain taxol cytotoxicity alone. Representing the first cellular barrier for anticancer agents, the plasma membrane is also probably engaged. Studying the interactions of taxol with various phospholipids will clarify whether it follows the route of other antineoplastic agents binding to nucleic acids and membranes59 or to other targets. In support of the role of biomembranes in taxol action mechanisms, using cell cultures appears to be the best approach as compared to intact animals where the conditions cannot be controlled sufficiently58. Thus, employing a variety of cell lines, more valuable data concerning lipid effects on neoplastic membrane enzymes, receptors, pumps and energy transducing systems can be obtained. For instance, to study the taxol cytotoxicity, both wild type and MDR animal cell cultures could be used. In this respect, a new hypothesis can be generated, according to which taxol could by-pass P-gp pathway and proceed by other routes, developing chemosensitivity. To test this model, chemosensitivity causing agents can be utilised. The observation of taxol accumulation upon their administration in P-gp defficient carcinoma cells, would prove this hypothesis. Such findings would indicate a P-gp independent pathway of action, leading to effects on chemoresistance and causing an increase in membrane permeability. To prove this, various taxol-biomembrane systems involving molecules leading to alterations in membrane fluidity and to increase in chemosensitivity (chemosensitizers) should be employed in a dose-dependent manner. This could be followed by applying for instance 1,6-diphenyl-1,3,5-hexatriene (DPH) and Laurdan fluorescence measurements in isolated tumor plasma membranes, in liposomes or in wild type or MDR animal and human cell membranes. This would allow studying membrane dynamics in both the outer hydrophobic region of the bilayer and in the acyl chain region, respectively. Thus, using such chemosensitizers acting by increasing lipid bilayer permeability would show correlations with anticancer cytotoxicity. In conclusion, a new model can be build, based on the action of chemosentisizers causing changes in membrane structures and leading to membrane fluidity fluctuations and increasing bilayer permeability, showing the significant role of these events in taxol cytotoxicity. Subsequently, new therapeutic strategies can be designed. Unfortunately, it is not yet possible to classify the effects of lipid composition on membrane function according to obtained patterns of taxol-lipid binding affinities. The obtained data is too fragmentary and too much depending on experimental conditions to permit generalizations. On the other hand, cell culture systems are too complicated, to be used satisfactorily regarding the mechanisms of action of taxol. More reliable approach would be the use of phospholipid vesicles, varying the lipid comosition and incorporating an exchange protein. Therefore, in our opinion, reasonable approach would be to use cultured cells and then extrapolate the built models and mechanisms to either a reconstituted system or to isolated membranes by incubation with lipid vesicles.
Besides these cellular effects, the subject of taxol-lipid interactions has also practical implications. The drug's poor aqueous solubility necessitates the application of various dispersions in its many commercial formulations, which unfortunately leads to acute hypersensitivity reactions or neuropathies besides other problematic issues30-33,38,40-44. A variety of mostly nanoscale strategies including nanoparticles, polyglutamates, co-polymers, prodrug and analog developments, vitamin E containing and other emulsions, microspheres and liposomes have been tried40-44. Liposomes provide less toxic tool for solubilizing the drug and increasing its therapeutic index in model tumor systems79,81. More data is needed for designing improved liposomal chemotherapeutic systems. Whether specific for drug-binding membrane lipid recognition domains exist and how they contribute to the affinity of drugs penetration into the lipid bilayer, as well as the importance of the membrane surfaces and curvatures are issues which remain to be defined. Undoubtedly, in terms of both cell biological and drug development issues, studying taxol-lipid interactions would help to elucidate its role in cellular toxicity and would open new opportunities for designing improved anticancer drug formulations.
CONCLUSIONS
Despite the significant improvements in cancer treatments, the resistance to a broad spectrum of structurally diverse chemotherapeutic drugs is of major concern. Growing research evidence depicts the importance of lipids in cancer and multidrug resistance. Thus, these molecular events occur after partitioning of the engaged glycoproteins into the lipid bilayer and changes in membrane physicochemical properties may have essential effects with important functional implications. The precise mechanisms of the involvement of tumor cell membrane in regulating anticancer drug-induced programmed cell death remains poorly understood. In this respect, studying the interactions of various antineoplastic agents with model and biomembranes attracts research efforts. The current work presents our recent measurements on the surface recognition between widely used chemotherapeutic agent taxol and phospholipids. The significance of the physical properties of the drug is emphasized. The basic applications of IR spectroscopy in following taxol-lipid interactions show promising results in terms of high signal-to-noise ratio, as well as for improvement of spectral resolution of the complex formations. The method can also be readily applied for studying pathologies in various cells and tissues.
REFERENCES
1. Daniel, R., Bumdan, Alan H. Calvert and Rowinski, EK., eds. Handbook of Anticancer Drug Development. Lippincott Williams & Wilkins, 2003, Baltimore, Maryland, USA.
2. Pommier, Y., Yu, Q and Kohn, KW. Chapter 2: Novel Targets in the Cell Cycle and Cell Cycle Checkpoints, In: Baguley, BC and Kerr DJ, eds. Anticancer Drug Development, 2002, Elsevier Inc.,13-30.
3. Hait, WN., Rubin, E and Bertino, JR. Chapter 48: Cancer Therapeutics. In: Mendelsohn, J., Howley, PM., Israel, MA., Gray, JW and Thompson, CB, eds. The Molecular Basis of Cancer, 3rd Edition, Saunders, Elsevier Inc. Philadelphia, PA, USA, 2008, 571-581.
4. Schultz, RM. Dawn of a New Era in Molecular Cancer Therapeutics. In: Schultz, RM, ed. Progress in Drug Research, Birkhäuser Verlag, Basel, Switzerland, 2005, 1-17.
5. Fischer, PM. Chapter 11: Cell Cycle Inhibitors in Cancer: Current Status and Future Directions. In: Neidle S. ed. Cancer Drug Design and Discovery, Elsevier Inc., 2008, 253-283.
6. Boyer, MJ and Tannock, IF. Chapter 17: Cellular and Molecular Basis of Drug Treatment for Cancer, In: Tannock, IF., Hill, RP., Bristow RG and Harrington, L. The Basic Science of Oncology, 4th Edition, The McGraw-Hill Companies, Inc., 2005, 349-375.
7. Ganansia-Leymarie V, Bischoff P, Bergerat JP, Holl V. Signal transduction pathways of taxanes-induced apoptosis. Curr Cancer Drug Targets 2003; 3 (3):193-03
8. Abal M, Andreu JM, Barasoain I. Taxanes: microtubule and centrosome targets, and cell cycle dependent mechanisms of action. Curr Cancer Drug Targets 2007; 7 (8): 713-29
9. Estève MA, Carré M, Braguer D. Microtubules in apoptosis induction: are they necessary? Curr Med Chem Anticancer Agents 2003; 3 (4): 291-06
10. Avendaño, C. and Menéndez, JC. Medicinal Chemistry of Anticancer Drugs. Chapter 8: Anticancer Drugs Targeting Tubulin and Microtubules, Elsevier B. V., 2008, 229-249.
11. Prislei S, Mozzetti S, Filippetti F, De Donato M, Raspaglio G, Cicchillitti L, Scambia G, and Ferlini C. From plasma membrane to cytoskeleton: a novel function of semaphorin 6A. Mol Cancer Ther 2008; 7 (1): 233-41
12. Straight AF, Field CM. Microtubules, membranes and cytokinesis. Curr Biol 2000; 10 (20): R760-70
13. Lane J, Allan V. Microtubule-based membrane movement. Biochim Biophys Acta 1998; 1376 (1): 27-55
14. Bloom GS, Goldstein LS. Cruising along microtubule highways: how membranes move through the secretory pathway. J Cell Biol 1998; 140 (6): 1277-280
15. Sheetz MP. Microtubule motor complexes moving membraneous organelles. Cell Struct Funct 1996; 21 (5): 369-73
16. Farsad K, De Camili P. Mechanisms of membrane deformation. Curr Opin Cell Biol 2003; 15 (4): 372-81
17. Petty, HR. Molecular Biology of Membranes-Structure and Function. Chapter 9: Membranes in Cancer. Plenum Pres, New York and London, 1993, 353-377
18. Harrison MR, Holen KD, Liu G. Beyond taxanes: a review of novel agents that target mitotic tubulin and microtubules, kinases, and kinesins. Clin Adv Hematol Oncol 2009; 7 (1): 54-4
19. van Meer G, Voelker DR, Feigenson GW. Membrane lipids: where they are and how they behave. Nat Rev Mol Cell Biol. 2008; 9 (2):112-24
20. Carpinteiro A, Dumitru C, Schenck M, Gulbins E. Ceramide-induced cell death in malignant cells. Cancer Lett 2008; 264 (1): 1-10.
21. Vance JE. Phosphatidylserine and phosphatidylethanolamine in mammalian cells: two metabolically related aminophospholipids. J Lipid Res. 2008 Jul;49(7):1377-87.
22. Hulbert AJ, Pamplona R, Buffenstein R, Buttemer WA. Life and death: metabolic rate, membrane composition, and life span of animals. Physiol Rev 2007; 87 (4): 1175-213
23. Grimm S, Brdiczka D. The permeability transition pore in cell death. Apoptosis 2007; 12 (5): 841-55
24. Moss DK, Betin VM, Soazig M, Lane JD. A novel role for microtubules in apoptotic chromatin dynamics and cellular fragmentation. J Cell Sci 2006; 119 (11) 2362-374
25. Croft DR, Coleman ML, Li S, Robertson D, Sullivan T, Stewart CL, Olson MF. Actin-myosin based contraction is responsible for apoptotic nuclear disintegration. J Cell Biol 2005; 168: 245-55.
26. Charras GT, Coughlin M, Mitchison TJ, Mahadevan L. Life and times of a cellular bleb. Biophys J 2008; 94 (5):1836-853.
27. Sheetz MP, Sable JE, Döbereiner HG. Continuous membrane-cytoskeleton adhesion requires continuous accommodation to lipid and cytoskeleton dynamics. Annu Rev Biophys Biomol Struct 2006; 35: 417-34.
28. van Blitterswijk WJ, van der Luit AH, Caan W, Verheij M, Borst J. Sphingolipids related to apoptosis from the point of view of membrane structure and topology. Biochem Soc Trans 2001; 29 ( 6): 819-24
29. Lindberg U, Karlsson R, Lassing I, Schutt CE, Höglund AS. The microfilament system and malignancy. Semin Cancer Biol 2008;18 (1): 2-11
30. Singla AK, Garg A, Aggarwal D. Paclitaxel and its formulations. Int J Pharm 2002; 235: 179-92
31. Stohs, SJ. Taxol in Cancer Treatment and Chemoprevention. In: Bagchi, D and Preuss, HG eds. Phytopharmaceuticals in Cancer Chemoprevention. CRC Press, Boca Raton, London, New York, Washington D. C., 2005, 519-524.
32. Hennenfent KL, Govindan R. Novel formulations of taxanes: a review. Old wine in a new bottle? Ann Oncol 2006;17: 735-49
33. Safavy A. Recent developments in taxane drug delivery. Curr Drug Deliv 2008; 5: 42-4
34. Wall ME, Wani MC. Camptothecin and taxol: discovery to clinic (13th Bruce F. Caine Memorial Award Lecture). Cancer Res 1995; 55:753
35. Wall ME, Wani MC. Camptothecin and taxol: from discovery to clinic. J Ethopharmacol 1995; 51:239
36. Harlan WR Jr. New opportunities and proven approaches in complementary and altenative medicine research at the National Institutes of Health. J Altern Compl Med 2001; 7: S53
37. Zefirova O, Nurieva E, Ryzhov A, Zyk N, Zefirov N. Taxol: Synthesis, Bioactive Conformations, and Structure-Activity Relationships in Its Analogs. Russ J Org Chem 2005; 41: 315-51
38. Sznitowska M, Lunder M, Placzek M. Paclitaxel solubility in aqueous dispersions and mixed micellar solutions. Chem Pharm Bull 2008; 56: 70-4
39. Seydel, JK and Wiese, M. Drug-Membrane Interactions. Analysis, Drug Distribution, Modeling. In: Mannhold, R; Kubinyi, H and Folkers, G eds. Methods and Principles in Medicinal Chemistry, Wiley-VCH Verlag GmbH, Weinheim, Germany, 2002, Vol. 15.
40. Balasubramanian SV, Straubinger RM. Taxol-lipid interactions: taxol-dependent effects on the physical properties of model membranes. Biochemistry 1994; 30: 8941-7
41. Castor TP. Phospholipid nanosomes. Curr Drug Deliv 2005; 4: 329-40
42. Nornoo AO, Osborne DW, Chow DS. Cremophor-free intravenous microemulsions for paclitaxel I: formulation, cytotoxicity and hemolysis. Int J Pharm 2008; 349: 108-16
43. Nuijen B, Bouma M, Schellens JH, Beijnen JH. Progress in the development of alternative pharmaceutical formulations of taxanes. Invest New Drugs 2001; 19: 143-53
44. Terwogt JM, Nuijen B, Huinink WW, Beijnen JH. Alternative formulations of paclitaxel. Cancer Treat Rev 1997; 23: 87-5
45. Günzler, H. and Gremlich, H-U. IR Spectroscopy. An Introduction, Wiley-VCH Verlag GmbH, 69469, Weinheim, Germany, 2002.
46. Lasch, P. and Kneipp, J. eds. Biomedical Vibrational Spectroscopy, John Wiley & Sons, Inc. Hoboken, New Jerswey, USA, 2008.
47. Coto X, Iloro I, Arrondo JLR. Two-dimensional infrared studies of biomolecules. In: Larijani, B; Colin AR and Woscholski R eds. Chemical Biology-Techniques and Applications, John Wiley & Sons, Chichester, UK, 2006, 151-161.
48. Diem M, Romeo M, Boydston-White S, Miljkovic M, Matthaus C. A decade of vibrational micro-spectroscopy of human cells and tissue (1994-2004). Analyst 2004; 129: 880-5
49. Andrus PG. Cancer monitoring by FTIR spectroscopy. Technol Cancer Res Treat 2006; 5: 157-67
50. Bloomfield VA, Crothers DM. Tinoco, I.Jr., Nucleic Acids-Structures, Properties, and Functions. University Science Books, Sausalito, CA 94965, 2000; 210-212.
51. Braun CS, Kueltzo LA and Middaugh CR. Ultraviolet absorption and circular dichroism spectroscopy of nonviral gene delivery complexes. In: Findeis, MS ed. Methods in Molecular Medicine, Vol. 65: Nonviral Vectors for Gene Therapy, Humana Books Inc., Totowa, NJ., 2001, vol. 65, 253-284.
52. Banyay M, Sarkar M, Gräslund A. A library of IR bands of nucleic acids in solution. Biophys. Chem 2003; 104: 477-88.
53. Galeotti T, Borrello S, Minotti G, Masotti L. Membrane alterations in cancer cells: the role of oxy radicals. Ann N Y Acad Sci 1986; 488: 468-80.
54. Dimanche-Boitrel MT, Meurette O, Rebillard A, Lacour S. Role of early plasma membrane events in chemotherapy-induced cell death. Drug Resist Updat 2005; 8: 5-14
55. Muller CP, Krueger GR. Modulation of membrane proteins by vertical phase separation and membrane lipid fluidity. Basis for a new approach to tumor immunotherapy. Anticancer Res 1986; 65: 1181-3
56. Deliconstantinos G. Physiological aspects of membrane lipid fluidity in malignancy. Anticancer Res 1987; 7: 1011-1
57. Campanella R. Membrane lipids modifications in human gliomas of different degree of malignancy. J Neurosurg Sci 1992; 36: 11-5
58. Baritaki S, Apostolakis S, Kanellou P, Dimanche-Boitrel MT, Spandidos DA, Bonavida B. Reversal of tumor resistance to apoptotic stimuli by alteration of membrane fluidity: therapeutic implications. Adv Cancer Res 2007; 98: 149-90
59. Rebillard A, Lagadic-Gossmann D, Dimanche-Boitrel M-T. Cisplatin cytotoxicity: DNA and plasma membrane targets. Curr Med Chem 2008; 15: 2656-3
60. Coates J. Interpretation of Infrared Spectra, A Practical Approach. In: Meyers, RA ed. Encyclopedia of Analytical Chemistry. John Wiley & Sons Ltd, Chichester, UK, 2000, 10815-7
61. Meyer JD, Manning MC, Carpenter JF. Effects of potassium bromide disk formation on the infrared spectra of dried model proteins. J Pharm Sci 2004; 93: 496-06
62. Choosakoonkriang S, Wiethoff CM, Anchordoquy TJ, Koe GS, Smith J, Middaugh CR. Infrared spectroscopic characterization of the interaction of cationic lipids with plasmid DNA. J Biol Chem 2001; 276: 8037-3
63. Clement J, Keifer K, Kimpfler A, Garidel P, Peschka-Süss R. Large-scale production of lipoplexes with long shelf-life. Eur J Pharmaceut Biopharm 2005; 59: 35-3
64. Liao P-C, Tan S-K, Lieu C-H, Jung, H-K. Involvement of Endoplasmic Reticulum in Paclitaxel-Induced Apoptosis. Journal of Cellular Biochemistry 2008; 104: 1509-23
65. Ganansia-Leymarie V, Bischoff P, Bergerat JP, Holl V. Signal transduction pathways of taxanes-induced apoptosis. Curr Med Chem Anticancer Agents. 2003; 3 (4): 291-06
66. Wang TH, Wang HS, Soong YK. Paclitaxel-induced cell death: where the cell cycle and apoptosis come together Cancer 2000; 88 (11): 2619-28
67. Wang LG, Liu XM, Kreis W, Budman DR. The effect of antimicrotubule agents on signal transduction pathways of apoptosis: a review. Cancer Chemother Pharmacol 1999; 44 (5): 355-61
68. Blagosklonny MV, Fojo T. Molecular effects of paclitaxel: myths and reality (a critical review). Int J Cancer 1999; 83 (2): 151-6
69. Fojo T, Menefee M. Mechanisms of multidrug resistance: the potential role of microtubule-stabilizing agents. Ann Oncol 2007; 18 (5): v3-v8
70. Longley DB, Johnston PG. Molecular mechanisms of drug resistance. J Pathol 2005; 205: 275-292
71. Al-Shawi MK, Omote H. The remarkable transport mechanism of P-glycoprotein; a multidrug transporeter. J Bioenerg Biomembr 2005; 37 (6): 489-496
72. Mary L. Membrane Structural Biology-With Biochemical and Biophysical Foundations. Cambridge University Press, New York, NY 10013-2473, USA, 2008; 296-308.
73. Borst P, Elferink RO. Mammalian ABC transporters in health and disease. Annu Rev Biochem. 2002; 71: 537-92.
74. Borgnia MJ, Eytan GD and Assaraf YG. Competition of hydrophobic peptides, cytotoxic drugs, and chemosensitizers on a common P-glycoprotein pharmacophore as revealed by its ATPase activity. J Biol Chem 1996; 271 (6): 3163-71
75. Eytan GD, Regev R, Assaraf YG. Functional reconstitution of P-glycoprotein reveals and apparent near stoichiometric drug transport to ATP hydrolysis. J Biol Chem 1996; 271 (6): 3172-78
76. Karavasilis V, Reid A, Sinha R, De Bono JS. Chapter 17: Cancer Drug Resistance. In: Neidle, S. ed. Cancer Drug Design and Discovery, Elsevier Inc., 2008, 405-23
77. Di Michele M, Della Corte A, Cicchillitti L, Del Boccio P, Urbani A, Ferlini C, Scambia G, Donati MB, Rotilio D. A proteomic approach to paclitaxel chemoresistance in ovarian cancer cell lines. Biochim Biophys Acta. 2009; 1794 (2): 225-36.
78. Regev R, Katzir H, Yeheskely-Hayon D., Eytan GD. Modulation of P-glycoprotein-mediated multidrug resistance by acceleration of passive drug permeation accross the plasma membrane. FEBS J 2007; 274: 6204-14
79. Huwyler J, Drewe J, Krähenbühl S. Tumor targeting using liposomal antineoplastic drugs. Intern J Nanomed 2008; 3 (1) 21-9
80. Baumert C, Hilgeroth A. Recent advances in the development of P-gp inhibitors. Anticancer Agents Med Chem 2009; 9 (4): 415-36
81. Gregoriadis, G. ed. Liposome Technology-Volume 2: Entrapment of Drugs and Other Materials into Liposomes, 3rd edition. Informa Halthcare USA, Inc., New York, NY 10016, USA, 2007.
82. Emuss V. and Marais R. Chapter 16: The Biology and Oncology of RAF-ERK Signaling. In: Neidle, S. ed. Cancer Drug Design and Discovery, Elsevier Inc., 2008, 382-402
83. Vance DE. Role of phosphatidylcholine biosynthesis in the regulation of lipoprotein homeostasis. Curr Opin Lipidol 2008; 19 (3): 229-34.
84. Yeagle, PL ed. The Structure of Biological Membranes. 2nd edition, CRC Press LLC, Boca Raton, London, New York, Washington DC., 2005.
85. Vigh L, Escribá PV, Sonnleitner A, Sonnleitner M, Piotto S, Maresca B, Horváth I, Harwood JL. The significance of lipid composition for membrane activity: new concepts and ways of assessing function. Prog Lipid Res 2005; 44 (5): 303-44.
86. Pilzer D, Gasser O, Moskovich O, Schifferli JA, Fishelson Z. Emission of membrane vesicles: roles in complement resistance, immunity and cancer. Springer Semin Immunopathol 2005; 27 (3):375-87.
87. Struchkov VA, Strazhevskaya NB. Structural and functional aspects of nuclear lipids in normal and tumor cells. Biochemistry (Mosc) 2000; 65 (5): 525-45
88. Lavie Y, Fiucci G, Czarny M, Liscovitch M. Changes in membrane microdomains and caveolae constituents in multidrug-resistant cancer cells. Lipids 1999; 34: S57-63
89. Eriksson LC, Andersson GN. Membrane biochemistry and chemical hepatocarcinogenesis. Crit Rev Biochem Mol Biol 1992; 27 (1-2):1-55
90. Schneiter MPR. Lipid signalling in disease. Nat Rev Mol Cell Biol 2008; 9 (2): 162-76
91. Silvius J. Lipid microdomains in model and biological membranes: how strong are the connections? Q Rev Biophys 2005; 38 (4): 373-83.
92. Jacobson K, Mouritsen OG, Anderson RG. Lipid rafts: at a crossroad between cell biology and physics. Nat Cell Biol 2007; 9 (1): 7-14.
93. Baumann N, Colsch B, Lefèvre M, Portoukalian J. Cellular lipid dynamics. J Soc Biol 2003; 197 (3): 205-10.
94. Borst P, Zelcer N, van Helvoort A. ABC transporters in lipid transport. Biochim Biophys Acta 2000; 1486 (1): 128-44.
95. Van Laethem F, Leo O. Membrane lipid rafts: new targets for immunoregulation. Curr Mol Med. 2002; 2 (6): 557-70.
96. Ali IU, Schriml LM, Dean M. Mutational spectra of PTEN/MMAC1 gene: a tumor suppressor with lipid phosphatase activity. J Natl Cancer Inst 1999; 91 (22): 1922-32.
97. Evans SV, Roger MacKenzie C. J Mol Recognit. Characterization of protein-glycolipid recognition at the membrane bilayer. 1999; 12 (3): 155-68.
98. Niemelä PS, Hyvönen MT, Vattulainen I. Atom-scale molecular interactions in lipid raft mixtures. Biochim Biophys Acta. 2009; 1788 (1): 122-35.
99. Janardhan S, Srivani P, Sastry GN. Choline kinase: an important target for cancer. Curr Med Chem. 2006; 13 (10): 1169-86.
100. Ramírez de Molina A, Rodríguez-González A, Lacal JC. From Ras signalling to ChoK inhibitors: a further advance in anticancer drug design. Cancer Lett 2004; 206 (2): 137-48.
101. Campbell RB, Balasubramanian SV, Straubinger RM. Influence of cationic lipids on the stability and membrane properties of paclitaxel-containing liposomes. J Pharm Sci 2001; 90 (8): 1091-05
102. Datta, DB. A Comprehensive Introduction to Membrane Biochemistry. Floral Publishing, Madison, WI, USA, 1987, 545-563.
103. Tan ML, Ooi JP, Ismail N, Moad AI, Muhammad TS. Programmed cell death pathways and current antitumor targets. Pharm Res. 2009; 26 (7): 1547-60.
104. Escribá PV. Membrane-lipid therapy: a new approach in molecular medicine. Trends Mol Med 2006; 12 (1): 34-43.
105. Escribá PV, González-Ros JM, Goñi FM, Kinnunen PK, Vigh L, Sánchez-Magraner L, Fernández AM, Busquets X, Horváth I, Barceló-Coblijn G. Membranes: a meeting point for lipids, proteins and therapies. J Cell Mol Med 2008; 12 (3): 829-75.
106. Sudhahar CG, Haney RM, Xue Y, Stahelin RV. Cellular membranes and lipid-binding domains as attractive targets for drug development. Curr Drug Targets 2008; 9 (8): 603-13.
107. Jendrossek V, Handrick R. Membrane targeted anticancer drugs: potent inducers of apoptosis and putative radiosensitisers. Curr Med Chem Anticancer Agents. 2003 Sep;3(5):343-53.
108. Fredman P, Hedberg K, Brezicka T. Gangliosides as therapeutic targets for cancer. BioDrugs. 2003;17(3):155-67.
109. Ferté J. Analysis of the tangled relationships between P-glycoprotein-mediated multidrug resistance and the lipid phase of the cell membrane. Eur J Biochem. 2000; 267 (2) :277-94
110. Blagosklonny, MV, Schulte T, Nguyen P, TrepelJ, Neckers LM., Taxol-induced apoptosis and phosphorylation of Bcl-2 protein involves c-Raf-1 and represents a novel c-Raf-1 signal transduction pathway. Cancer Res 1996; 56: 1851-54
111. Okano J-i., Rustgi AK. Paclitaxel induces prolonged activation of the Ras/MEK/ERK pathway independently of activating the programmed cell death machinery. J Biol Chem 2001; 276 (22), 19555-64
112. Data SR, Brunet A, Greenberg ME. Cellular survival: a play in three Akts. Genes Dev 1999; 13: 2905-27
113. Misra S, Ujházy P, Varticovski L, Arias IM. Phosphoinositide 3-kinase lipid products regulate ATP-dependent transport by sister of P-glycoprotein and multidrug resistance associated protein 2 in bile canalicular membrane vesicles. Proc Natl Acad Sci U S A 1999; 96 (10): 5814-9.
114. Yang C. Upregulation of caveolin-1 and caveolae organelles in Taxol-resistant A549 cells. FEBS Lett 1998; 439 (3): 368-72
115. Carre M, Andre N, Carles G, Borghi H, Brichese L, Briand C, Braguer D. Tubulin is an inherent component of mitochondrial membranes that interacts with the voltage-dependent anion channel. J Biol Chem 2002; 277: 33664-69
116. Pushkarev VM, Starenki DV, Saenko VA, Namba H, Kurebayashi J, Tronko MD, Yamashita S. Molecular mechanisms of the effects of low concentrations of Taxol in anaplastic thyroid cancer cells. Endocrinology 2004; 145 (7): 3143-52
117. Hendrich AB, Michalak K. Lipids as a target for drugs modulating multidrug resistance of cancer cells. Curr Drug Targets 2003; 4 (1): 23-30
ACKNOWLEDGEMENTS:
This work was supported by Gazi University Research Fund (Project N°: 02-2005/15). The author greatly acknowledges facilities and personnel of The Central Laboratory of Middle East Technical University (Ankara-Turkey) for access to instruments.
CORRESPONDENCE:
Dr. Erhan Süleymanoglu
Gazi University. Faculty of Pharmacy.
Dept. of Pharmaceutical Chemistry.
Hipodrom 06330.
Ankara. Turkey
Mail: esuleymanoglu @ gazi.edu.tr
Comment of the reviewer Amélie Rebillard PhD. Centre de Lutte contre le Cancer Paul Papin. Centre de Recherche Inserm Nantes-Angers. Angers. France.
The authors describe an interesting method for studying interactions between membrane and chemotherapeutic drugs. Infrared spectroscopy open new possibilities to design novel antitumor drug formulations with better bioavailability properties and less toxic side effects.
Comment of the reviewer Prof. Ahmad Safavy PhD.Associate Professor. Department of Radiation Oncology and the Comprehensive Cancer Center. University of Alabama at Birmingham. Birmingham, AL. USA
This article introduces a new approach to the illustration of drug-cell membrane (D-CM) interactions by employing infrared (IR) spectroscopic techniques. The authors have selected an important anticancer drug, paclitaxel (taxol), which despite its impressive antitumor and apoptotic activity, could lead to significant drug resistance in treated patients. Given the importance of D-CM interactions in the formation of drug resistance and induction of apoptosis, a new approach for studying such interactions is a welcome event. Furthermore, some added advantages of this technique are expected to be its simplicity and ease of experimentation, as well as being economically practical.
Received: February 15, 2009. Received reviewed: July 27, 2009
Published September 20, 2009